Fig. 3.1
Early ischemic CT signs. Noncontrast CT images show early ischemic changes, loss of insular ribbon at the right side (a), loss of lentiform nucleus at the left side (b), and hyperdense artery sign (arrow) at the right MCA M1 distal segment (c)
Early signs of ischemia are often subtle on NCCT, also having a high intra- and interobserver variability. Additionally, it can be challenging to identify early ischemic changes within chronic white matter hypodensities. The NCCT signs of ischemia during the first 3 h of symptom onset have a sensitivity of 26–60% and a specificity of 85%; the positive and negative predictive values are 96% and 27%, respectively [12, 13]. In the setting of lacunar infarcts and hypodense changes in the posterior circulation, NECT has a lower sensitivity [14].
Alberta Stroke Program Early CT Score (ASPECTS)
The extent of early ischemic change may be differently determined among reviewers because early ischemic change is often difficult to determine. The ASPECTS is a simple and systematic approach to quantifying the extent of irreversibly damaged parenchymal hypoattenuation on pretreatment CT scans in patients with acute ischemic stroke of the MCA (Fig. 3.2) [15]. Although the presence of early ischemic changes on CT, regardless of their extent, is not a contraindication to IV-tPA treatment, it has been shown that detecting early ischemic changes within the early period of AIS is important for determining a patient’s prognosis and complications [16–18]. ASPECTszz 0–5 indicates exclusion of patients from endovascular treatment because of its futility [18]. However, some patients with ASPECT <5 can benefit from endovascular treatment [16]. Thus, it is still unknown whether such patients should be excluded or not. However, the current AHA/ASA guidelines for acute stroke management list a frank hypointensity (infarct) of greater than one-third of the MCA territory on NCCT as an imaging exclusion criterion for IV t-PA [6]. Additional advantage of ASPECTS is that it combines a semiquantitative estimate of volume along with localization. It weighs smaller volumes in the basal ganglia and internal capsule equally with larger volumes of brain designated M1 through M6. This approach is useful because lesion volume alone on NCCT is only weakly correlated with neurological outcome [19]. The ASPECT score is used more and more and has even been applied to DWI. The ASPECTS, however, has some issues that remain to be resolved: One is no anatomic landmarks for distinction of each M region and another is that the interobserver reliability of each region on CT is relatively low (mean intraclass correlation coefficients were 0.640 in M1–M3; 0.530 in M4–M6; 0.762 in the insula, lentiform nucleus, and caudate; and 0.367 in the internal capsule) [20].


Fig. 3.2
Alberta Stroke Program Early CT score (ASPECTS). The levels of thalamus and basal ganglia and most superior margin of the ganglionic structures ASPECTS slices show normal 10-point areas. Left hemisphere, ASPECTS study form: C caudate head, L lentiform nucleus, IC internal capsule, I insular ribbon, MCA middle cerebral artery, M1 anterior MCA cortex, M2 MCA cortex lateral to insular ribbon, M3 posterior MCA cortex; M4, M5, and M6 are anterior, lateral, and posterior MCA territories, respectively, approximately 2 cm superior to M1, M2, and M3, respectively, rostral to basal ganglia
3.2.2 Computed Tomography Angiography
Stroke mimickers such as seizures, metabolic abnormalities, and neuropsychiatric conditions may be difficult to distinguish from acute cerebral ischemia on purely clinical grounds. Thus, an objective method for confirming intracranial vessel occlusions prior to treatment is highly required. This method should be rapid, easily interpretable, be relatively independent of operator skills, and have the potential for widespread availability. CT angiography (CTA) may play an important role in answering these questions. CTA offers the possibility to visualize the location, extent, and aspect of an arterial occlusion, may reveal important information about the collateral circulation, and depicts the extracranial vessels from the aortic arch [21–23]. The addition of CTA over NCCT significantly increased diagnostic sensitivity and likelihood of treatment with tPA in patients with acute stroke [24].
3.2.2.1 Assessment of Vascular Occlusion
In CT angiography (CTA) iodinated contrast is administered intravenously. The scanning typically starts from the aortic arch up to a level above the circle of Willis when the contrast medium is in the arterial phase. The new generation of CT scanners can reconstruct a CT digital subtraction angiography where bone and soft tissue are subtracted, visualizing only the vasculature (Fig. 3.3). Stenoses and occlusions can be detected with high diagnostic accuracy in both extracranial and intracranial arteries thus revealing the origin of the AIS (the mean sensitivity, specificity, PPV, NPV, and accuracy were 83.2% (95%, CI 57.9–100%), 95.0% (95%, CI 74.4–100%), 84.1% (95%, CI 50.0–100%), 97.1% (95%, CI 94.0–100%), and 94.0% (95%, CI 83.0–99.0%), respectively [25]. CTA is a rapid, easily available imaging tool for detecting intracranial occlusions in patients with acute ischemic stroke, thereby guiding therapy (Fig. 3.4) [26].



Fig. 3.3
CT angiography – bone subtraction angiography. AP view (a) and lateral view (b) CTA MIP (maximum intensity projection) images well visualized intracranial arteries

Fig. 3.4
Intracranial CT angiography (a) demonstrates occlusion (arrow) of left superior division of MCA M1. Intracranial and extracranial CT angiography (b) shows occlusion (arrow) of left MCA M1 distal segment with visualization of distal MCA branches beyond occlusion site
3.2.2.2 Assessment of Collateral Vascular Status
An acute intracranial occlusion produces an area of ischemic penumbra, the extent being dependent on residual and the collateral blood flow [27–29]. In contrast to digital subtraction angiography, CTA lacks information about the flow of contrast from the arteries via capillaries to the veins. Despite this, CTA can provide a relevant overview of collateral flow, including leptomeningeal collaterals around the ischemic area. This information may be valuable in predicting the probability of salvaging brain tissue. Now we have used leptomeningeal collateral grading system using CTA in anterior circulation stroke (Table 3.1). The poor grade CT collateral which was measured by variable collateral grading system was an independent predictor of extremely poor outcomes [30].
Table 3.1
Leptomeningeal collateral grading system using CT angiography
rLMCa | 0–20 point | 0 = no visible collateral flow | 0–10 poor | SCTA |
11–16 medium | ||||
20 = collateral flow equal to or stronger than in unaffected hemisphere in all regions | 17–20 good | |||
Miteffb | 3-point | 1 = when contrast opacification is merely seen in distal superficial branches | Poor | SCTA |
2 = vessels can be seen at Sylvian fissure | Medium | |||
3 = if vessels are reconstituted distal to occlusion | Good | |||
PAFSc | 6-point | 0 = when compared with the asymptomatic contralateral hemisphere, there are no vessels visible in any phase within the ischemic vascular territory | Poor | MCTA |
1 = there are just a few vessels visible in any phase within the occluded vascular territory | Poor | |||
2 = there is a delay of two phases in filling in of peripheral vessels and decreased prominence and extent or a one-phase delay and some ischemic regions with no vessels | Poor | |||
3 = there is a delay of two phases in filling in of peripheral vessels or there is a one-phase delay and significantly reduced number of vessels in the ischemic territory | Poor | |||
4 = there is a delay of one phase in filling in of peripheral vessels, but prominence and extent is the same | Intermediate | |||
5 = there is no delay and normal or increased prominence of pial vessels/normal extent within the ischemic territory in the symptomatic hemisphere | Good |
Single-phase CT angiography does not have temporal resolution; therefore, collateral status may be mislabeled in many patients [31]. A multiphase or dynamic CTA has been shown to be better in predicting clinical outcome in acute ischemic stroke patients compared to single-phase CTA by more accurately depicting the state of collateral vessels [32]. Dynamic CT angiography is a technique that derives time-resolved images of pial arterial filling from perfusion CT images; however, it needs postprocessing and whole-brain perfusion CT (Fig. 3.5) [33, 34].


Fig. 3.5
Leptomeningeal collateral grading using dynamic CTA. (a–d) Good collateral grade. (a) A proximal right ICA occlusion on maximum intensity projection (MIP) CTA. Temporal maximum intensity projection (tMIP) images (b–d) using perfusion CT show well backfilling arteries at the right MCA arteries. (e–h) Poor collateral grade. (e) A proximal right ICA occlusion on MIP CTA. tMIP images (f–h) using perfusion CT show minimal backfilling of right MCA arteries
3.2.2.3 Detection of Ischemic Change
Hypoattenuation on CTA source image (CTA-SI) is an indicator of reduced cerebral blood volume in the area of ischemia. Numerous studies have also shown that CTA-SI improves the prediction of final infarct size and clinical outcome when compared to NCCT [36–39].
However, this is the case only when CT angiography (CTA) is obtained with relatively slower scanners. Recent scanners with multi-detector rows can obtain arterial phase images much faster than old generation scanners, resulting in larger poor contrast-filling areas in cases of major artery occlusion, which may overestimate infarct core [40–42]. CTA-SI, thus, is not a reliable tool to identify infarct core when it is obtained with faster scanners.
3.2.3 Computed Tomography Perfusion
The practical advantages of CTP are that it is widely available and does not delay treatment decisions because it is fast and most patients already undergo CT scanning [43].
3.2.3.1 Assessment of Ischemic Penumbra
The goal of CT perfusion imaging (CTP) is to assess the ratio of infarct core (irreversibly damaged brain tissue) to penumbra, thus identifying the “tissue at risk.” In hypoperfused areas of brain parenchyma, there are typically high MTT values due to supply via collateral circulation. Autoregulation attempts to preserve CBF values by inducing vasodilatation, which results in an increased CBV. When ischemic injury is more severe and prolonged, autoregulation is unable to maintain the CBV above the threshold for neuronal death, and the tissue experiences irreversible hypoxic damage with a subsequent decrease in CBV; this area represents the infarct core, where tissue no longer viable will not benefit from reperfusion and is at risk for hemorrhage (Fig. 3.6). CTP has caused confusion with regard to the definition of infarct core. At first, an absolute cerebral blood volume (CBV) value of 2.0 mL/100 g was adopted to determine the infarct core [44–46]. Subsequently, it was suggested that a relative cerebral blood flow (rCBF) <31% threshold best determines infarct core [47]. However, other studies indicate that the most reliable predictor of infarct core is the reduction of the CBF to 30–50% relative to the mean CBF in the contralateral hemisphere [44, 45, 48].


Fig. 3.6
(a) MIP CTA shows occlusion of the right MCA M1 segment. (b) Decreased CBV at the right lentiform nucleus, (c) large area of delayed TTP lesion is visualized at the right MCA territory, (d) delayed MTT lesion area is similar to TTP lesion, and (e) right MCA territory has decreased CBF. (f) Follow-up 1 day DWI shows acute infarcted lesion at the right lentiform area. This lesion is similar to CBV lesion in terms of size and location
Operational Infarct Core Threshold in CTP
- 1.
Absolute CBF of <12 mL/100 mg/min with CBF ratio of <32% and absolute CBV of <2 mL/100 g with CBV ratio of <68%
The penumbra is the critically hypoperfused tissue, which is still viable if reperfusion is achieved. It is of great interest to differentiate the penumbra from benign oligemia. Outside the penumbra, there is an area of benign oligemia which is hypoperfused to a lesser degree; it remains viable even if reperfusion fails. In that sense, “ischemic penumbra” and “tissue at risk” are interchangeable terms. The penumbral region the levels were 12–25 mL/100 g/min in PET study (oligemic or not at-risk tissue, was also identified by PET and is defined as a region with reduced CBF, increased OEF, and normal CMRO2) [49]. There are several approaches for estimating the penumbra on CTP. The most common one is to presume that areas with solely reduced CBF and/or TTP/Tmax are considered to show the penumbra [44, 45, 50]. Other penumbra threshold has been reported to exist on MTT or Tmax maps [46, 47, 51, 52].
Operational Penumbra Threshold in CTP
- 1.
CBF <20 mL/100 g/min with Tmax of 5.5 s
- 2.
CBV >2 mL/100 g with MTT >145% to contralateral side
- 3.
CBF 18–37 mL/100 g/min; MTT 1.8–8.3 s relative to the contralateral side [111]
- 4.
TTP >5 s to contralateral side
The visible mismatch between the volume of tissue with decreased blood flow (i.e., delayed TTP, MTT, Tmax and/or low CBF) and that with significantly low CBV can be used as a CTP-based operational definition of the ischemic penumbra [53–55]. CTP provides equivalent results to diffusion/perfusion MRI in terms of characterizing the infarct and penumbra (Fig. 3.7) [40, 46, 47].






Fig. 3.7
A 91-year-old woman with right-sided weakness and facial palsy (NIHSS 12). She visited ER 60 min after symptom onset. Before IV-tPA therapy, noncontrast CT (NCCT) images (a) and CT perfusion (4D CTA) (b, c) were done. NCCT images (a) show hyperdense artery sign (arrow) at the right MCA M1 distal segment (right) and subtle early ischemic sign (loss of lentiform nucleus) (middle). (b) MIP CTA shows occlusion of right proximal MCA M1. (c) CT perfusion maps show small-sized CBV lesion and large time perfusion parameters (TTP/MTT). (d) Probability model map of using TTP + 6.8 s (penumbra, yellow zone) and rCBV −41% (infarct core, red zone) (ventricle, purple zone). (e) Dynamic CTA shows retrograde filling of MCA M3, M2 branches (arrows) by leptomeningeal collateral flow. (f) After stroke imaging, the patient received IV-tPA with no improvement in symptoms. The patient has a large penumbra area and small infarct core; it means it is a good candidate of endovascular therapy. After endovascular therapy, MRA shows complete recanalization (right), and diffusion-weighted image shows the infarcted area where the probability map showed. Immediately following endovascular therapy, the patient demonstrated clinical improvement (NIHSS 4)
3.2.3.2 Techniques of CT Perfusion
CT perfusion (CTP) or MR perfusion (MRP) is a technique where the same area of the brain is repeatedly scanned during the passage of the contrast medium from the arteries through the capillaries to the veins and then into the venous sinuses [13, 56]. Although there are important technical differences between modalities, both CT and MR perfusion imaging are based on the same mathematical models as positron emission tomography (PET). Therefore, the overall clinical applicability of perfusion imaging by using either of these techniques is similar. The most important advantage of CTP is the linear relationship between contrast concentration and attenuation in CT, which facilitates quantitative (versus relative) measurement of CBF and CBV. MR perfusion imaging (MRP) relies on the indirect T2* effect induced in the tissue by gadolinium; the T2* effect itself is not linearly related to the gadolinium concentration, making absolute measurement of CBF and CBV difficult [56].
CTP involves dynamic acquisition of sequential CT slices during rapid IV administration of iodinated contrast material. CTP allows rapid, noninvasive, quantitative evaluation of cerebral perfusion. Based on the multi-compartmental tracer kinetic model, dynamic CTP imaging is performed by monitoring the first pass of an iodinated contrast agent bolus through the cerebral circulation. Because the change in CT density (in Hounsfield units) is proportional to the concentration of contrast, perfusion parameters are calculated by deconvolution from the changes in the density-time curve for each pixel using mathematical algorithms based on the central volume principle [57, 58]. Deconvolution-based algorithms allow much lower injection rates, 5 mL/s as reported earlier, compared with algorithms that use other approaches, such as the nondeconvolution-based maximal slope model [56]. These lower injection rates are more practical and tolerable for patients. For the quantification of perfusion parameters, arterial input function (AIF) should be measured. The ACA or middle cerebral artery (MCA) can be selected as the AIF. A large venous structure such as the torcular herophili is chosen as the output vein. Examination of the venous contrast concentration versus time curve assists in the normalization of the resulting perfusion parameters by helping to correct for partial volume averaging effects. For this reason, analysis of perfusion CT data using the deconvolution method requires the selection of two small regions of interest (ROIs) reflecting representative time–attenuation curves for the arterial input function and venous outflow function [56, 59].
Calculated CT perfusion maps include the following:

- 1.
Mean transit time (MTT) indicates the average time between arrival of the bolus in a given tissue and the outflow of the bolus from the tissue into the venous system also measured in seconds.
- 2.
Cerebral blood volume (CBV) indicates the total volume of blood in the intravascular space of each tissue, including capillaries and large vessels, measured in milliliters of blood/100 g of brain tissue (normal range in gray matter, 4–6 mL/100 g).
- 3.
Cerebral blood flow (CBF) is the volume of blood flowing through a brain tissue per unit time, measured in milliliters of blood/100 g of brain tissue/minute (normal range in gray matter, 50–60 mL/100 g/min).
- 4.
The relationship between CBF and CBV is expressed by the equation CBF = CBV/MTT.
- 5.
Tmax indicates time-to-maximum of the flow-scaled residue function.
- 6.
Additional perfusion parameters:
Time-to-peak (TTP) is an estimate of the time between arrival of the tracer bolus at the precapillary arteriole and peak concentration of tracer in the capillary bed, measured in seconds.
First moment (FM) is the centroid of the area under the curve (AUC) of the time-concentration curve onto the time axis.
Bolus arrival time (BAT) of the time-concentration curve (the curve c voi (t) does not start to rise at the same time point as c art (t) starts to rise). The difference between these two time points can be defined as the bolus arrival time (BAT) (Fig. 3.8).

Fig. 3.8
Perfusion parameters that are measured using the time-concentration curve (a) and flow-scaled residual function curve (b). BAT bolus arrival time, TTP time to peak, FM first moment, Tmax time-to-maximum of the flow-scaled residue function, rCBV relative cerebral blood volume
3.3 Magnetic Resonance Imaging
A multimodal magnetic resonance imaging (MRI) protocol for AIS patients should be short and effective aiming to show acute ischemic areas, exclude hemorrhage, and also provides identifying the location and extent of intravascular clot as swell as the presence and extent of penumbra. Also, multimodal magnetic resonance imaging (MRI) is useful for determining treatment strategies in the acute stage. In the acute stage, early diagnosis of ischemic stroke and its differentiation from stroke-mimics are important [60, 61]. Although CT is the most commonly used modality for stroke imaging, because of its wide availability and faster acquisition time, some comprehensive stroke centers choose stroke MRI rather than CT for two major reasons. First is higher sensitivity and specificity of MR imaging for detection of hyperacute ischemia. Diffusion-weighted imaging (DWI) provides the most specific way to image acute infarction. The advent of MRI has redefined stroke syndromes such as acute ischemic infarction and TIA. Second is the absence of radiation.
Comprehensive MR stroke protocols have four “p” components: (1) parenchymal imaging, which identifies the presence and size of an irreversible infarcted core, determines presence of hemorrhage, and helps to age the ischemic event; (2) pipes, MRA/CE-MRA to determine the location of arterial occlusion and susceptibility-weighted imaging (SWI/T2*-weighed imaging) to detect presence of an intravascular thrombus that can be treated with thrombolysis or thrombectomy; (3) penumbral imaging to determine the presence of hypoperfused tissue at risk for subsequent infarction if adequate perfusion is not restored; (4) perfusion imaging to determine sum total CBF arriving at a particular brain region both via normal route and recruited collaterals [62].
3.3.1 Diffusion-Weighted Imaging
Diffusion-weighted imaging (DWI) detects cytotoxic edema due to restricted water diffusion in brain tissue [63]. DWI has an excellent sensitivity in the detection of acute ischemia compared to CT [64, 65] and shows areas of cytotoxic edema as soon as 3–11 min after onset of AIS [66].
DWI is significantly more sensitive and specific in the identification of early ischemic brain injury (sensitivity 91–100%, specificity 86–100%), far beyond NCCT [67–69]. As such, most lesions with diffusion restriction are generally considered irreversible in clinical practice. There are reports of DWI lesions being reversible especially after mechanical thrombectomy [70]. These initially reversible lesions on an early MR (performed 24–48 h after therapy) may reappear if imaging is repeated 1–2 weeks later [71]. The higher the apparent diffusion coefficient value is, the higher the chance is that the lesion is reversible [72]. Complete reversal of DWI lesions after reperfusion is limited to tiny lesions in embolic stroke patients [73].
Diffusion lesion volume (infarct volume) has become a more important factor to predict clinical outcome. In the anterior circulation, a large infarct with cytotoxic edema on DWI involving more than one-third of the MCA territory, an infarct volume >100 mL is associated with poor clinical outcome [12, 71, 72]. Also some studies suggest that patients with DWI lesion >70 mL [74] or >100 mL [75] do not benefit from endovascular treatment due to futility. In this patient group, reperfusion therapy is not recommended. DWI lesion size may help guide therapeutic decision-making between surgical decompression and recanalization therapy. However, the exact threshold of DWI lesion volume to exclude patients from endovascular treatment has yet to be determined because some patients with larger DWI lesion volumes had favorable outcomes [76, 77].
3.3.2 Susceptibility-Weighted Imaging
Acute stroke imaging requires the differentiation between ischemic stroke and hemorrhagic stroke. A T2*-weighted gradient-echo image or susceptibility-weighted imaging (SWI) is reported to be as accurate as CT in the detection of hyperacute hemorrhage and superior to CT for chronic hemorrhages [78, 79]. SWI can detect acute subarachnoid hemorrhage [80] and is extremely sensitive for subacute and chronic hemorrhage that is not demonstrated with FLAIR or CT [81].
SWI or T2*-weighted image can be used to identify acute thrombus in a similar way to that of unenhanced CT [82] in acute ischemic stroke. The information regarding the presence of intra-arterial thrombus and its location may be useful in planning various treatment options. Intra-arterial thrombus makes the “susceptibility vessel sign” and is defined as the presence of hypointensity within the arteries in which the diameter of the hypointense vessel exceeded the contralateral vessel diameter (Fig. 3.9) [83]. Susceptibility vessel sign was an independent predictor of cardioembolic stroke and subsequent recanalization [84]. However, it is often limited because of the following reasons: First, it may overestimate thrombus extent by dark signal intensity from stagnating blood distal to occlusion. Second, it is prone to artifact, which is problematic at the skull base. Last, it may not be helpful to characterize thrombus [85]. SWI can also demonstrate prominent asymmetrical cortical and transmedullary veins in the region of ischemia, which possibly represent the region of increased oxygen extraction fraction (Fig. 3.9).


Fig. 3.9
Susceptibility vessel and transverse medullary vein signs. (a) Noncontrast CT shows hyperdense artery sign (arrow) at the right MCA M1 segment. (b) MRA shows occlusion of right MCA M1. Susceptibility-weighted image (SWI) shows dark susceptibility vessel sign (arrow) corresponding to CT clot (c) and transmedullary vein sign (arrows) on ventricular level (d). After complete recanalization of occlusion, transmedullary vein sign is disappeared on SWI (e)
Hemorrhagic transformation of ischemic stroke can be a devastating complication especially if the patient is considered for revascularization therapies. It is observed in approximately 20–40% of all stroke patients within the first week of onset [86]. Conventional MRI or NCCT often does not detect these hemorrhagic transformations, especially hemorrhagic infarction type 1 or 2. SWI which is exquisitely sensitive to hemorrhage is able to detect small hemorrhages within the infarct and makes it more conspicuous than T2*-weighted image.
SWI is significantly more sensitive for detection of chronic intracerebral microbleeds, which may be the sequelae of amyloid angiopathy or chronic hypertension. It may be difficult, however, to differentiate smaller acute hemorrhages from older hemosiderin deposits, and their presence is a much disputed contraindication for thrombolysis [48]. But, the risk of hemorrhage in patients with more than five chronic microhemorrhages is unknown [87].
SWI can provide complementary information in stroke patients in the form of:
- 1.
Identification of hemorrhagic transformation of an ischemic infarct occurring due to arterial or venous thrombus.
Stay updated, free articles. Join our Telegram channel
Full access? Get Clinical Tree
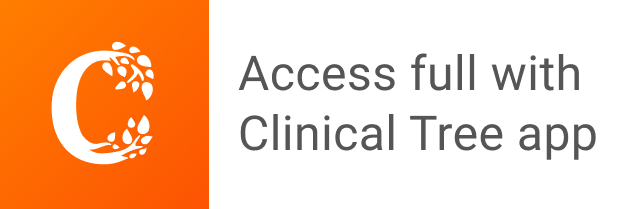