Respiratory Anatomy and Physiology
David F. Wolfe
LEARNING OBJECTIVES
On completion of this chapter, the reader should be able to:
1. Identify the anatomic name and location of each portion of the respiratory system from the mouth to the blood flowing through the lungs including the relevant respiratory muscles and their control.
2. Discuss the physics of respiratory gas exchange, the role of oxygen and carbon dioxide concentration gradients, and the thin membranes involved in gas exchange.
3. Describe several aspects of the relationship between ventilation and perfusion of the lungs, including anatomy, gas exchange, and neural/chemical control of ventilation.
4. Describe the general effects of sleep on respiratory and cardiovascular control of ventilation.
5. Describe the differential effects of waking, nonrapid eye movement sleep, and rapid eye movement sleep on ventilation and heart rate.
6. Identify and describe the more common pathologic states associated with breathing control mechanisms including central apnea/hypopnea, obstructive apnea/hypopnea, treatment-emergent central sleep apnea, Cheyne-Stokes respiration, and periodic breathing.
KEY TERMS
Alveoli
Aortic bodies
Apnea
Atrium
Bicarbonate
Carbon dioxide (CO2)
Carbonic acid
Carbonic anhydrase
Carotid bodies
C-fiber receptors
Chemoreceptors
Cheyne-Stokes respiration
Concentration gradients
Cranial nerves
Dead space
Diaphragm
Diffusion
Expiratory reserve volume
Functional residual capacity
Hypercapnia
Hypopnea
Hypoxia
Inspiratory capacity
Inspiratory reserve volume
Intercostals
Intrapleural space
Larynx
Mediastinum
Medulla
Obstructive sleep apnea (OSA)
Oxygen (O2)
Oxyhemoglobin dissociation curve
Parietal pleura
Pharynx
Phrenic nerve
Pneumocytes
Pneumothorax
Pons
Pulmonary capillaries
Rapidly adapting receptors
Residual volume
Respiration
Shunt
Slowly adapting receptors
Surfactant
Tidal volume
Total lung capacity
Trachea
Tricuspid valve
Vena cava
Ventricle
Visceral pleura
Vital capacity
Respiration is, in all animals, a vital process responsible for the delivery of oxygen (O2) to the tissues to support metabolism and the removal of the metabolic waste gas, carbon dioxide (CO2). The major mechanisms of this exchange of gases between the external and internal environments are (1) simple passive diffusion along concentration gradients and (2) transport of these gases by the circulatory system. Concentration gradients refer to the difference in the concentration of a gas or liquid across a semipermeable barrier. In very small animals, such as single-celled organisms, gaseous diffusion occurs across their cell membranes, but larger organisms have distances that are too great for passive diffusion. Animals evolved a small number of very effective solutions, for example, a closed circulatory system and highly vascularized lungs or gills. Lungs (or gills) provide a specialized structure for placing a large amount of circulating body fluid (e.g., blood) very close (<1 mm) to a large amount of environmental media (e.g., air). Proximity across a permeable membrane that separates different concentrations of O2 and CO2 allows rapid movements of both gases down their concentration gradients, normally in opposite directions.
In higher chordate animals, respiratory transfer of CO2 and O2 is a two-step, cyclic process. The first step is at the interface between the body tissues (e.g., muscle cells, liver cells) and the circulating lung capillary blood. This step is characterized by O2 movement from blood plasma across the thin separating membrane that makes up the capillary wall and into a metabolizing tissue (e.g., muscle or brain). Concurrently, the CO2 liberated by tissue metabolism diffuses from the metabolizing cells across the tissue capillary membrane and into the circulating blood.
The second step occurs in the lungs between the lungs’ airspaces and the circulating blood in very small capillaries within the lungs. Opposite from the tissue part of the cycle, gas movements in the lung are characterized by O2 movement from the air within the lung into the pulmonary capillary blood, whereas CO2 moves from the capillary blood into the minute air spaces called “alveoli” within the lung. The next expiration then expels most of the CO2, and the next inspiration replenishes the O2 supply. This two-part process is termed “gas exchange.” The transfers of metabolic gases are passive processes because both gases are simply moving down their concentration gradients at both the pulmonary and the tissue locations.
Necessary and complementary components of this process are the circulation of blood and ventilation of the lungs. Therefore, the heart and blood vessels are essential components of respiration and are intimately involved in blood gas homeostasis. Breathing control mechanisms exist to control the concentrations of O2 and CO2 in the lung air and, subsequently, blood within the lung, which is carried to the tissues to support normal metabolism. In other words, the controls of respiratory movements are mostly based on sensory signals from the brain and body chemoreceptors, sensing O2 levels, and CO2/pH levels. These sensory signals mostly come from chemosensitive neurons in the brainstem, especially the medulla, the carotid bodies in the neck, and the aortic bodies on the aortic arch. The carotid and aortic bodies are chemosensitive to O2 and CO2/pH. These signals then “feed back” to the brainstem controllers, which then reduce hypercapnia (an excess of CO2) or hypoxia (a deficiency of O2 in the airways) by increasing ventilation and cardiac output. This is a classic example of a negative feedback loop used to control some critical physiologic variable. These respiratory actions are well coordinated with the cardiovascular system to achieve effective blood gas homeostasis.
In humans and other mammals, the respiratory drive to ventilate the lungs is mostly performed by neural networks in the brain. In fact, much of the brainstem and significant portions of certain “higher” areas of the brain are devoted to respiratory and cardiovascular control, especially cardiopulmonary coordination. Although purely physical and biochemical factors can and do affect respiration, by far, most mechanisms for maintaining and adjusting pulmonary ventilation are found in the brainstem and spinal cord. As a first step into the mechanisms used to control gas exchange, we review the structural components of the system, because a basic biologic principle is the intricate interdependence between form (anatomy) and function (physiology).
A REVIEW OF RESPIRATORY-RELATED ANATOMY AND PHYSIOLOGY
Any comprehensive discussion of respiratory function requires a review of thoracic, circulatory, and central nervous system (CNS) structure. Respiration and respiratory control encompass many anatomic and physiologic features that interact to meet very specific goals of homeostasis. One excellent example of this interaction is that of the circulatory and respiratory systems. The O2 content and the CO2 content within the circulatory system are the focus of the homeostatic controllers of ventilation.
Upper Respiratory Tract
Air drawn into the nasal passages and oral cavity is humidified, filtered, and warmed (in cooler climates) at the beginning of the pathway (see Fig. 7-1). Humidification of the incoming air is complete, resulting in pulmonary airspaces with 100% water saturation. The inside surfaces of the oral cavity and tongue contribute to water saturation of the incoming breath and
also collect particulates from the incoming air, using surface water and turbulent airflow. In addition to humidification, the mucus membranes of the irregularly shaped turbinates within the nasal passages promote turbulence in the airflow, which assists the filtering of particulates. Air turbulence serves to increase filtering efficiency because small particles in the inhaled, turbulent air are more likely to eventually contact a mucus membrane than would particles within a straight streamlined airflow. Nasal hairs also serve to create turbulence in the nasal airflow to maximize the filtering and humidification of the inspired air. These hairs also screen out large particles in the air stream. The captured particles (dust, ash, pollen, skin flakes, etc.) are then swallowed, coughed out, or blown out of the nose by a sneeze. The main utility of this physical process is that the amount of particulate matter going into the lung is minimized.
also collect particulates from the incoming air, using surface water and turbulent airflow. In addition to humidification, the mucus membranes of the irregularly shaped turbinates within the nasal passages promote turbulence in the airflow, which assists the filtering of particulates. Air turbulence serves to increase filtering efficiency because small particles in the inhaled, turbulent air are more likely to eventually contact a mucus membrane than would particles within a straight streamlined airflow. Nasal hairs also serve to create turbulence in the nasal airflow to maximize the filtering and humidification of the inspired air. These hairs also screen out large particles in the air stream. The captured particles (dust, ash, pollen, skin flakes, etc.) are then swallowed, coughed out, or blown out of the nose by a sneeze. The main utility of this physical process is that the amount of particulate matter going into the lung is minimized.
![]() Figure 7-1 A schematic of the respiratory system with the most important anatomic features emphasized. Note the narrowing of the airspace around the pharynx and larynx. |
Just behind the nasal passages and the oral cavity is the pharynx, essentially the upper part of the throat. As suggested by Figure 7-1, nasal and oral air mix here before passing into the larynx; this portion of the upper airway is located between the pharynx and the trachea and contains the vocal cords. The pharynx is a common site for airway collapse or partial obstruction, especially during supine sleep. The collapse may lead to a complete occlusion, called an apnea, or a partial collapse allowing some airflow, called a hypopnea. Muscles of the larynx and pharynx respond to sleep like most other skeletal muscles, with a significant decrease in muscle tone and reactivity (1). Most of the upper airway is surrounded by pharyngeal and laryngeal muscles that are important in airway rigidity, airway size, coughing, and speech. A bolus of food or water activates complex laryngeal motor reflexes, which guide the bolus into the esophagus, away from the vocal cords and trachea.
The pharynx and the larynx are the usual sites for obstructive ventilatory events; that is, both structures tend to collapse and occlude the airway. One common scenario is when the back of the tongue relapses onto the posterior walls of the pharynx. The relapse is often caused by insufficient tongue muscle activation during sleep, especially in supine sleep and obesity. This is the classic description of obstructive sleep apnea (OSA), or obstructive hypopnea. The insufficiency is partly related not only to the normal decrease in motor tone during sleep, but also to anatomic features, such as the tongue’s size and mass and/or micrognathia (small, recessed chin). The most important aspect of obesity in relation to obstructive apnea is when the area under the chin is obese and flaccid, allowing the tissue to sag into the mouth cavity leading to obstructions (2). Obviously, supine sleep significantly exacerbates the obstruction. In that position, the tissue sags toward the back of the throat, increasing the effect of an obstruction in the airway.
Other airway obstructions often occur because of sleep-related relaxation of the pharyngeal and/or laryngeal muscles, allowing negative airway pressure to pull the posterior wall of the airway forward toward the tongue and the front side of the airway. This obstruction usually creates either an apnea or a hypopnea. Airflow through the vocal cords and trachea is low resistance and generally unremarkable. This continuum from the mouth and nares to the superior end of the trachea represents the upper respiratory tract.
Lower Respiratory Tract
The lower respiratory tract begins at the upper end of the trachea (Fig. 7-1); this then connects to the pulmonary trunk that soon branches into the right and left bronchi (Fig. 7-2). At the first branching level, the trachea splits into right and left bronchi. The left bronchus branches into two smaller sections, whereas the right bronchus branches into three. The lung tissue supplied by these five bronchi corresponds to the five lobes of the lung: three lobes of the right lung and two lobes of the left lung. Branching continues, ending with the alveoli and the alveolar ducts. These levels are where gas exchange takes place.
The walls of the alveoli are composed of pulmonary epithelial cells and pulmonary capillaries carrying deoxygenated venous blood. There are two important cell types lining the alveolus: type I pneumocytes and type II pneumocytes. Type I cells make up the cell membrane or walls. The less numerous type II cells are the source of a substance called “surfactant,” which reduces surface tension on the inner alveolar walls. The reduced (and
variable) surface tension stabilizes the alveolus, making collapse less likely (3). The narrow divide (0.5 µm) between air in the alveoli and venous blood in the capillaries greatly facilitates gas exchange across the alveolar membrane with the nearby red blood cells (RBCs).
variable) surface tension stabilizes the alveolus, making collapse less likely (3). The narrow divide (0.5 µm) between air in the alveoli and venous blood in the capillaries greatly facilitates gas exchange across the alveolar membrane with the nearby red blood cells (RBCs).
When a reduced RBC (depleted of O2) reaches the alveolar membrane, the venous O2 pressure is approximately 40 mm Hg and the alveolar air, half a micron away, is about 100 mm Hg. This concentration gradient moves alveolar O2 easily across the membrane into the blood plasma and then into the RBC. The PO2 value for the exiting capillary blood, on its way to the left atrium of the heart, is approximately 150 mm Hg. In contrast, the CO2 pressure is about 45 mm Hg in venous blood and about 40 mm Hg in oxygenated alveoli, a relatively small concentration gradient. In healthy people, the transfer of that 5 mm Hg of CO2 takes about one-fourth second (similar to the time for RBC oxygenation), well before the RBC exits the pulmonary capillary (˜0.75 seconds).
Vascular Anatomy of the Lungs and Heart
It should be clear that the cardiovascular system is intimately involved in breathing control mechanisms. The flow of blood generated by the heart is how O2 reaches the tissues and how CO2 gets to the alveoli to be expired. There is a considerable symmetry between the vascular structure and the airway structure in the lower reaches of the lungs. This is necessary to bring the smallest airways and the very small pulmonary capillaries into close contact while maintaining sufficient blood pressure to keep the small vessels open and sufficient airway pressure to keep the most vulnerable airways open.
Venous blood from the body reaches the heart through two large veins: the superior vena cava and the inferior vena cava. The superior vena cava carries blood from the head, arms, and upper torso; the inferior vena cava carries blood from the legs and lower torso. These two large veins converge on the right atrium. During the part of the cycle when the cardiac muscle is relaxed, blood flows through the right atrium via the tricuspid valve and pools in the right ventricle (see Fig. 7-3). When the ventricle is full, systole (cardiac muscle contraction) begins with contraction of the right atrium, forcing atrial venous blood into the already-full ventricle. This stretches the ventricular muscle, raising the ventricular blood volume and pressure. The tricuspid valve between the right atrium and the right ventricle closes just as the right ventricle contracts. Contraction of the right ventricle expels the venous blood, forcing it through the pulmonary valve of the heart into the pulmonary artery and, eventually, the pulmonary capillaries of the alveoli within the lung. For the now-oxygenated blood exiting the alveoli, it is the same process back to the heart. The pulmonary capillaries come together to form very small veins, which merge with others to form a somewhat larger vein and so on. Finally, this O2-saturated blood travels the pulmonary vein back to the left atrium of the heart. As with the right side, the left atrium is essentially a primer pump to create sufficient intraventricular pressure to stretch the cardiac muscle and maximize the efficiency of the ventricular contraction. The left ventricle then expels its volume of oxygenated, low CO2 content blood into the arterial circulation (see Fig. 7-3).
The circulatory structure of the cardiopulmonary system is the one place in the body where venous blood is carried in an “artery,” because it is exiting the right ventricle but is still deoxygenated and hypercapnic (an excess of CO2). Similar to the airway conduction system, the large pulmonary artery coming from the right ventricle enters the lung and branches down to the level of the alveolar ducts and alveoli. Once the venous blood is into the pulmonary capillaries surrounding an alveolus, the CO2 within that blood flows down its concentration gradient, across the pulmonary membrane, into the alveolar air. At the same time, inspired O2 moves down its concentration gradient, across the alveolar membrane into the “venous” blood in the pulmonary capillaries, changing it to oxygenated arterial blood.
Thoracic Anatomy and Function
The main muscles of inspiration are the diaphragm and the external intercostals. Both are common skeletal muscles controlled by spinal motor nerves. The diaphragm and the external intercostals act in concert to create negative intrapulmonary pressure, resulting in airflow into the trachea, or inspiration. During an inspiration, ambient air enters the body through the nares, mouth, or both. Then, the air stream passes through the many branches of the airways, eventually reaching the vicinity of many alveoli. Diffusion moves the O2 the rest of the way into the alveoli and their capillaries.
The air within the pathways from the mouth and nares down to the terminal airways constitutes a significant volume that is not involved in an exchange of O2 and CO2. This is because air is in the passageways of the lung, not in the portions involved in gas exchange. This is the “conduction” portion of the respiratory system. The “respiratory” portion of the lungs is composed of the alveoli, alveolar ducts, and respiratory bronchioles. These are the levels at which gas exchange occurs. The airway conduction system is graphically demonstrated in Figure 7-2.
One of the two respiratory “pump” muscles is the diaphragm, necessary for normal ventilation, although many other muscles assist the diaphragm in producing ventilation. Ventilation is the physical consequence of normal cyclic respiratory muscle activity. Diaphragmatic contraction, due to CNS activation, causes the thoracic cavity floor to descend, making the thoracic cavity larger and creating negative pressure to draw in air from the environment, that is, inspiration. The diaphragm has almost no involvement in expiring air from the lungs. The other major inspiratory pump muscles are the external intercostals, which connect adjacent ribs. During inspiration, the external intercostals contract to pull the chest wall outward and upward to increase thoracic cavity size, contributing to the decrease of intrapulmonary pressure and making the chest wall stiffer (less compliant). There are also several sets of secondary, or accessory, inspiratory muscles. Accessory respiratory muscles include those in the abdomen, neck, pharynx, larynx, nose, tongue, and upper chest.
In polysomnography, changes in abdominal and thoracic circumference are usually recorded to assess ventilatory effort. This technique is actually measuring the displacement because of the external intercostals—diaphragmatic contraction does not increase chest circumference. This is still useful because it provides a relatively easy index of respiration, without complicated and invasive procedures or imaging.
In normal “at-rest” ventilation, expiration is entirely passive. Because during normal breathing no expiratory muscle activity is required, cyclic inspiration is the emphasis of the control system at rest. Of course, active expiration by expiratory muscles is often necessary, such as in exercise, to more quickly expel the breath and allow for appropriately rapid ventilation. The main expiratory muscles are the internal intercostals and abdominal muscles, but others also assist. Expiratory pump muscles act to increase intrathoracic pressure in order to increase intrapulmonary pressure and push air out of the lungs more quickly.
The conducting airways, from the mouth and nose to the lungs, are not involved in gas exchange, but are surrounded by both skeletal and smooth muscles that act to control the flow of air to and from the lungs (Fig. 7-2). Muscles of the upper respiratory tract are especially relevant in sleep medicine because of their involvement in OSA. The usual site of an obstruction is an area of the upper airway where muscles are relatively flaccid, or abnormally large. Muscles of the pharynx, larynx, and tongue make up this group. These too are skeletal muscles and, like the other accessory respiratory muscles, have functions other than ventilation—for example, swallowing, speech, and movement of the head, neck, or shoulders. Airway muscles are activated in a specific phase of respiration (inspiration or expiration), but they are not responsible for controlling intrathoracic pressures and, therefore, are not respiratory pump muscles.
OSA/hypopnea arises from insufficient compensation by muscular control systems for certain anatomic and postural features or deficiencies, such as the chin and neck obesity described earlier. This insufficiency is often not due to any change in the CNS controllers or blood gas reflexes, but simply due to the weight of the tongue (or tonsils) and the lack of physical support while sleeping supine. Sleep is a critical factor in apneas/hypopneas because neural output to all skeletal muscles, except the diaphragm, is reduced during sleep (4). OSA is an excellent illustration of the interaction between structure and function and of the impact of the neurophysiologic state, sleep, on those interactions.
The lungs themselves have no muscles for movement and are not physically attached to the chest wall; instead, an alternate mechanism evolved to couple the lungs to the thoracic wall. The outer surface of the lungs is called the “visceral pleura.” The visceral pleura also covers the mediastinum, a midline “structure” between the lungs that includes the trachea, heart, great vessels, thymus, and esophagus. The inside surface of the thoracic cavity is lined by a layer of epithelium called the “parietal pleura.” The visceral pleura is held to the parietal pleura by negative intrapleural pressure. It is important to realize that there are no anatomic features that hold the lungs against the inner thoracic walls. This physical coupling of the two pleura, resulting from the negative intrapleural pressure, ensures that the volume of the lungs varies directly with the size of the thoracic cavity. The pleura are mostly impervious to gases, helping maintain the negative pressure. Because there is normally almost no “space” in the “intrapleural space” (2 to 5 mL), it is often referred to as a “potential space.” Negative intrapleural pressure results from elastic recoil of the respiratory muscles and lungs and the surface tension of the aqueous film lining the inner alveolar surface. Elasticity is a structural property of lung tissue. Emphysema reduces elastic recoil through the destruction of alveolar walls and their pulmonary capillaries (5). The resulting enlargement of that airspace represents a greatly reduced surface area for gas exchange.
The term “pneumothorax” is used to convey a loss of negative pressure in the intrapleural space because of an opening to the atmosphere or into the lung. When the negative pressure is lost, the two pleura separate and air flows into the newly enlarged space. This effectively uncouples the close adhesion between inspiratory chest wall movement and lung expansion, that is, the lung collapses. A pneumothorax may occur spontaneously or as a result of trauma involving an opening in either of the pleura. For example, a stab or gunshot wound that penetrates both pleura can allow air from the atmosphere into the intrapleural space and the lung, removing the negative pressure gradient. This is a medical emergency requiring immediate assistance.
In patients without penetrating trauma of the chest wall, the diagnosis is usually based on the presence of chest pain, increased heart rate, tachypnea, hypoxemia, and shortness of breath. X-rays are also helpful in diagnosing a pneumothorax. Acute pneumothorax is usually treated with a needle aspiration of the intrapleural space and/or a chest tube. Both procedures require hospitalization. Other treatments include supplemental O2 and positive pressure ventilation. A chronic spontaneous pneumothorax can occur in the lung, often near the apex, and creates a hole in the visceral pleura, allowing lung air to flow into the negative pressure of the intrapleural space.
Innervation of the Respiratory Muscles and Blood Vessels
The diaphragm and external intercostals are driven by specific motor nerves from the spinal cord. The diaphragm is the main controller of ventilatory movements and is driven by the phrenic nerves. The external intercostals are the most relevant to respiratory control mechanisms. The output carried by the phrenic nerve is from the bilateral phrenic areas of the spinal cord gray matter. The spinal phrenic area is, in turn, controlled from specific areas in the brainstem. Neural control is addressed later in this chapter.
In addition to the inspiratory muscles, there are important expiratory muscles as well. The most important expiratory muscles are the internal intercostals and the abdominal muscles. Like the external intercostals, the internal intercostals are driven by nerves that emanate from the full length of the thoracic segment of the spinal cord. Motor nerves to the abdominal muscles derive from the lumbar portion of the spinal cord.
Blood vessel diameter, and subsequently pressure, is actively controlled by the sympathetic nervous system. The main output nuclei controlling cardiovascular sympathetic neurons are located in the brainstem and hypothalamus; these areas are driven by many inputs from several brain areas. The parasympathetic system has no known significant impact on the control of blood pressure. The other major controlling variable is the heart rate. The intrinsic heart rate is derived from the sinoatrial (SA) node, located in the right atrium. This “baseline” rate is normally modulated by many factors but mostly by the sympathetic system and the parasympathetic system. Usually, sympathetic excitation is associated with increased heart rate, enhanced cardiac muscle contractility (force of contraction), increased motor tone throughout the body, and often an increase in blood pressure. The parasympathetic effects are mostly the opposite of the sympathetic effects.
RESPIRATORY PHYSIOLOGY
Respiratory function is based on the need to acquire O2 for normal metabolism and to remove the metabolic waste gas, CO2. A paradox of this physiologic mechanism is that the respiratory system does not try to eliminate all of the CO2 in the lungs. Instead, the neurally mediated respiratory control system actively maintains CO2 at approximately 40 mm Hg. The respiratory system is an important component of acid-base (pH) balance within the body. Within RBCs, the combination of CO2 and water (H2O) into carbonic acid (H2CO3) is catalyzed by carbonic anhydrase. Blood plasma does not have this important enzyme. Carbonic acid dissociates
to hydrogen (H+) and bicarbonate ions (HCO3–). Maintaining CO2 at approximately 40 mm Hg results in an O2 pressure lower than it would be without the high level of CO2, illustrating the importance of the role played by the respiratory system in acid-base balance. Figure 7-4 shows the effects of various agents on the oxyhemoglobin dissociation curve, including acidity and PCO2: decreased PCO2 or increased pH shifts the curve to the left, decreasing PO2 for a given hemoglobin (Hb) saturation (increasing the Hb’s affinity for O2). Most relevant to the patient is where he or she is on the x-axis when the controlling factors are expressed (↑PCO2, ↓pH).
to hydrogen (H+) and bicarbonate ions (HCO3–). Maintaining CO2 at approximately 40 mm Hg results in an O2 pressure lower than it would be without the high level of CO2, illustrating the importance of the role played by the respiratory system in acid-base balance. Figure 7-4 shows the effects of various agents on the oxyhemoglobin dissociation curve, including acidity and PCO2: decreased PCO2 or increased pH shifts the curve to the left, decreasing PO2 for a given hemoglobin (Hb) saturation (increasing the Hb’s affinity for O2). Most relevant to the patient is where he or she is on the x-axis when the controlling factors are expressed (↑PCO2, ↓pH).
In what follows, there are many descriptions of gradients and concentration differences. Often, it is important to know the quantity of some gas, mixed with other gases and contained within some space (like a lung, or the earth’s atmosphere). The quantitative measure of that specific gas is the partial pressure (Table 7-1), conventionally in millimeters of mercury (mm Hg). The total of all partial pressures in the earth’s atmosphere is the barometric pressure, approximately 760 mm Hg. The partial pressure of O2 in our atmosphere is 158 mm Hg. Because 158 divided by 760 equals 0.208, the O2 in our atmosphere is approximately 21%. Almost all the rest is nitrogen, with a partial pressure of 596 mm Hg (78.4% of barometric pressure). Also, there is usually water in the atmosphere, with some obvious exceptions. A generalized value for the partial pressure of water in the atmosphere is 5.7 mm Hg or 0.008%. Therefore,
Pbaro = PO2 + PN2 + PH2O = 759.7.
Table 7-1 Partial Pressures (mm Hg) | ||||||||||||||||||||||||
---|---|---|---|---|---|---|---|---|---|---|---|---|---|---|---|---|---|---|---|---|---|---|---|---|
|
There is a very small amount of other gases in the atmosphere, such as argon (0.93%) and CO2 (0.03%). Still more gases are also present in air, but at very low concentrations.
The following is an illustrative example of a gradient: if the partial pressure of O2 in arterial capillary blood is 62 mm Hg and the partial pressure of O2 in an adjacent metabolizing cell is 35 mm Hg, then the O2 gradient is 27 mm Hg, from blood to tissue cells. The steeper the gradient, the greater is the force or speed of gas movement.
Respiratory Volumes and Capacities
There are several “compartments” within the respiratory system: first, the body trunk cavity lined with parietal pleura, then the thoracic cavity, then the lungs themselves within the visceral pleura, the upper airway, the trachea, the conducting portion of the bronchial tree, and, finally, the cavities within alveoli and alveolar ducts. These compartments comprise the pulmonary structure.
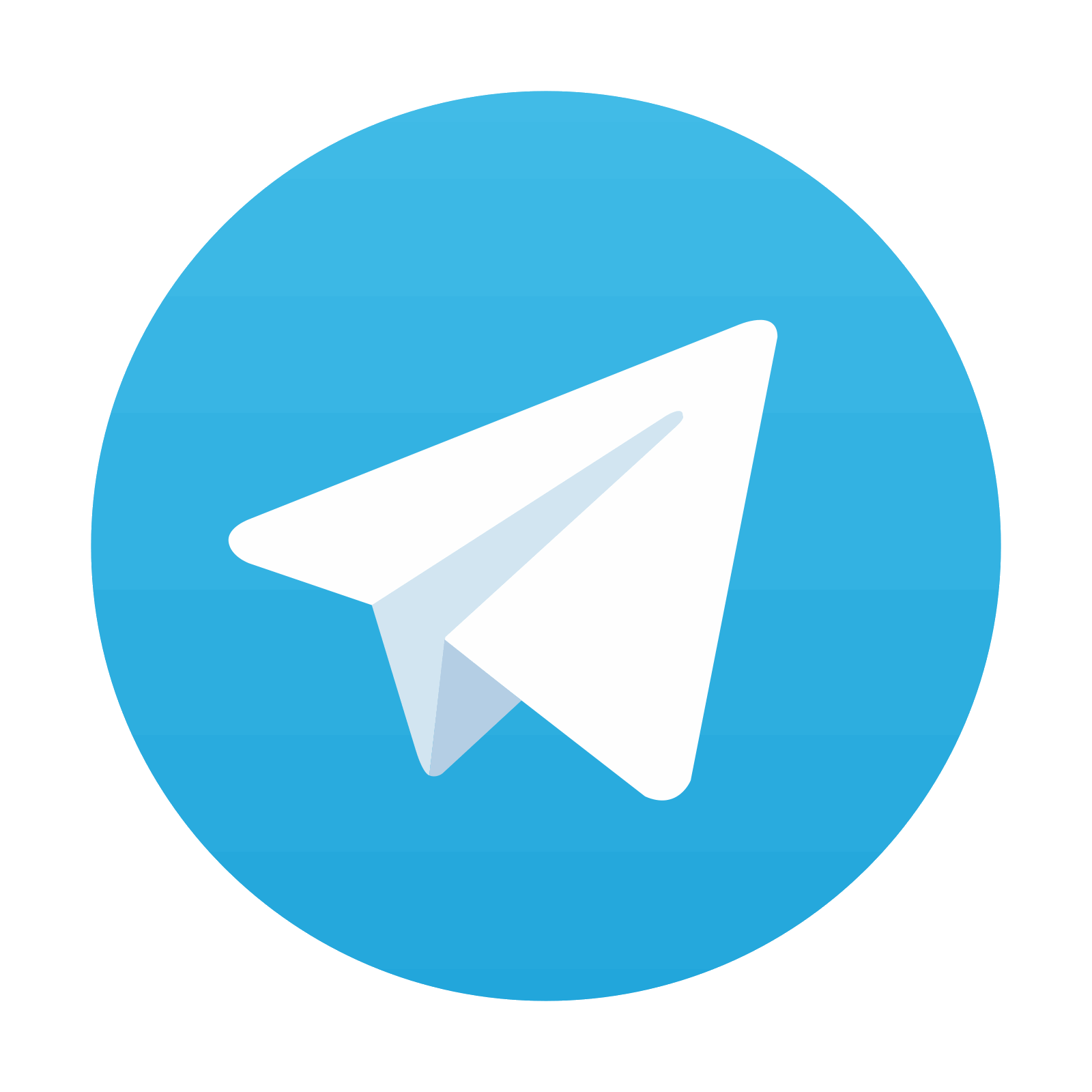
Stay updated, free articles. Join our Telegram channel
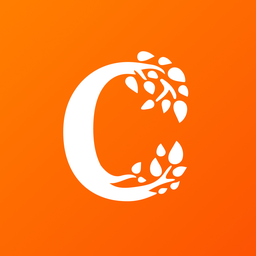
Full access? Get Clinical Tree
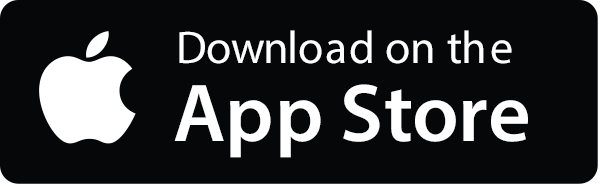
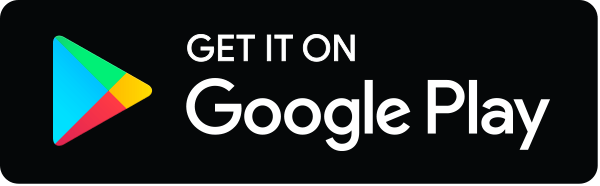