Fig. 8.1
Polygraphic recordings of Cheyne-Stokes respiration in a patient with congestive heart failure in 180 s. From top to bottom, electrocardiogram, oronasal flow, ribcage motion, and arterial oxygen saturation on pulse oximeter are displayed. A cyclical fluctuation with episodes of central apneas/hypopneas alternating with episodes of hyperventilation in a gradual waxing and waning fashion is seen

Fig. 8.2
Polygraphic recordings of Cheyne-Stokes respiration in a patient with congestive heart failure in 60 s. Displayed parameters are seen in Fig. 8.1. A clustering of heartbeats during hyperventilation phase and a scattering during apnea/hypopnea phase are seen
The CSR of an oscillatory ventilation has been the focus of investigation among physicians for more than a century [4–7], since its first description by Cheyne (1777–1836) and Stokes (1804–1878) in a heart failure patient [8], and it has been assumed that the underlying mechanism of CSR might be an unstable feedback control of the respiratory system [9–11]. However, the roles of the interacting respiratory/circulatory centers and, possibly, the vagal nerve contributing to the genesis of the CSR and, thereby, the Entrainment with CSR are still unknown.
The authors previously discussed the fundamental question of “Why does the heartbeat synchronize with respiratory rhythm?” to conclude that “RSA or heart rate variability in synchrony with respiration is a biological phenomenon, which may have a positive influence of gas exchange at the level of the lung via efficient ventilation/perfusion matching” [12]. This concept could be applicable to the Entrainment with CSR, and hence, the author has recently provided the concept that the efficacy of pulmonary gas exchange might be improved by the Entrainment with CSR, the phenomenon of which seems analogous to the RSA [3].
In this article, the authors are proposing the further concept that “RSA and the Entrainment with CSR are not simply secondary products of other known reflexes but have physiological roles with the cardiopulmonary works to be minimal by synchronizing heartbeats with breathing,” and we are reviewing how this teleological concept has been developed.
For this purpose, after showing the “Comparative Biology of Synchronizing Heartbeats with Breathing (8.2),” we are addressing the “Clinical/Physiological Significances of RSA, CSR, and Entrainment with CSR (8.3, 8.4, 8.5, 8.6).” In the “Physiological Significance of RSA (8.4),” we are summarizing our previous five separate experiments in canines (#1–#5 Experiments) along with their implications, respectively. Since the “Physiological Significances of CSR and Entrainment with CSR (8.6)” have been seldom investigated, our descriptions are hypothetical.
Herein, the authors are showing the perspectives of the RSA and the Entrainment with CSR according to our laboratory experiments and clinical experiences. This review article is written from standpoint of the wisdom of the body in maintaining the homeostasis efficiently [13], which can be achieved by synchronizing heartbeats with breathing [3, 12, 14], and consequently by saving the working loads of breathing [15] and circulation [16].
8.2 Comparative Biology of Synchronizing Heartbeats with Breathing
In the comparative biology, a cardiorespiratory interaction analogous to the RSA and the Entrainment with CSR is widely observed among many species of vertebrates from mammals, birds, and reptiles to water-breathing fish [17].
In mammals, the dog has a prominent RSA [18–20], and the human fetus obtains the RSA activity after a gestational age of ~33 weeks, and the healthy-term newborn infant has a strong RSA, which becomes a major component of heart rate variability in humans [21].
Regarding the Entrainment with CSR, the details of this phenomenon have been described, to our knowledge, only in humans with congestive heart failure [2, 3, 22]. However, a respiration-related oscillation in heartbeats, analogous to the RSA and the Entrainment with CSR, is observed in spontaneous breathing ducks [23], reptiles [24, 25], and fish. For example, in resting fish, gills are ventilated with pulsatile water flow throughout the respiratory cycle and heartbeat occurs in 1:1 synchrony with the respiration so that it results in coincidence of the periods of maximal flow rate of blood and water at the gills [26, 27]. This cardiorespiratory synchronization in fish is mediated by the vagal nerve and abolished by atropine [28].
The above observations among species suggest that the RSA and the Entrainment with CSR may bear a primitive role to maintain the homeostasis effectively by synchronizing heartbeats with breathing, although the role of the vagal nerve, especially, in the genesis of the Entrainment with CSR has been unknown.
8.3 Clinical Significance of RSA
A number of studies have been published concerning the mechanisms of RSA, and it has been known that RSA occurs mainly as the result of fluctuation of vagal outflow to the heart, despite the fact that the sympathetic outflow also influences the variability [29]. The RSA might result from modulation of vagal efferent activity to the sinus node through gating of the excitatory input to the vagal motor neurons from the lung inflation afferents [30]. During inspiration, impulses arising from the stretch receptors of the lungs are transmitted through the vagal nerve to inhibit the cardioinhibitory area in the medulla. As the tonic vagal discharge to keep the heart rate slow decreases, the heart rate increases. The degree to which this modulation occurs is a function of the level of tonic vagal discharge; thus, RSA measures would offer an index of cardiac vagal outflow [31, 32], under the most physiological circumstances [14].
The magnitude of RSA is clinically assessed as the amplitude of the high frequency band (HF band) of fluctuation of the R-R intervals (0.15 > Hz), utilizing the frequency analysis of heart rate variability. For accurate assessment, an examinee’s tidal breathing should be standardized due to the frequency-dependent characteristics of RSA [33]. Therefore, the amplitude of the HF band, i.e., the magnitude of RSA, has been considered to reflect the cardiac vagal activity, and it has been used as the index of the activity of the parasympathetic nervous system [34, 35]. Previous studies have shown that the RSA activity of “cardiac age” is most exaggerated in young and healthy humans, and the activity generally weakens in the geriatric population [36] and among patients with cardiovascular/pulmonary diseases, diabetes mellitus, and other systemic diseases [12]. The authors have already revealed the possible dissociation between the respiratory cardiac modulation (magnitude of RSA) and the cardiac vagal tone (vagal control of heart rate) in twofolds [14]. One is the bradycardia caused by the pharmacological baroreceptor stimulation [37], and the other is the magnified RSA without changes in heart rate and blood pressure caused by the chemoreceptor stimulation with hypercapnia [38, 39], the latter of which is discussed in Sect. 4.4.
8.4 Physiological Significance of RSA
8.4.1 Pulmonary Gas Exchange and RSA (#1 Experiment)
The physiological significance of RSA had been generally unknown until the late twentieth century, when the authors demonstrated experimentally that the efficacy of pulmonary gas exchange is improved by RSA in an animal model study [40]. Herein, we summarize the authors’ experiment on the RSA and pulmonary gas exchange in anesthetized canines [40], the species of which has the strong RSA [18–20].
8.4.1.1 Summary
In anesthetized canines with tracheal intubation, the sympathetic activity was eliminated with the premedication of reserpine, and the endogenous vagal activity was blocked with the left-side cervical vagotomy and right-side local anesthesia at the skull base. The right vagal nerve, innervating the sinus node, was electrically stimulated to control the frequency and timing of heartbeats. The artificial negative pressure ventilation was maintained with the diaphragm pacing (electrophrenic respiration) [41], since the mechanical positive pressure ventilation should reverse the intrathoracic pressure from negative during tidal breathing to positive, reducing the phasic increase of venous return to the thoracic cage during inspiration [42, 43] and affecting the features of RSA [44, 45].
The RSA model with heartbeats clustered during inspiration and scattered during expiration, the inverse-RSA model with heartbeats scattered during inspiration and clustered during expiration, and the control model with regular heartbeats with the identical R-R intervals were generated with the electrical stimulation to the right vagal nerve. Arterial/mixed venous blood and inspiratory/expiratory airflow were sampled for gas analyses. The fraction of alveolar gas volume unable to interface with sufficient blood flow during inspiration (wasted ventilation or the ratio of physiological dead space to tidal volume) [46] and the fraction of capillary blood volume unable to interface with sufficient fresh gas during expiration (wasted blood flow or the fraction of intrapulmonary shunt) [47] were compared among the three models.
As the result, these two parameters reflecting the lack of efficacy in the pulmonary gas exchange were minimal in the RSA model, maximal in the inverse-RSA model, and intermediate in the control model.
8.4.1.2 Implications
The results have suggested that the RSA of a respiratory-circulatory interaction benefits the pulmonary gas exchange and enhances the energy efficacy of circulation by supplying “necessary” heartbeats during inspiration and saving “unnecessary” heartbeats during expiration.
The concept that the efficacy of pulmonary gas exchange is improved by RSA, which could be applicable to the healthy humans [39–49], seems to have gained favor among pulmonary/cardiovascular physicians and anesthesiologists [50, 51]. However, controversy still remains on the effects of RSA on the pulmonary gas exchange [52–54], and the central or peripheral origins of RSA have been under much debate among physiologists [55, 56].
8.4.2 Respiratory Muscles and RSA (#2 Experiment)
We summarize the author’s experiment on the respiratory muscles and RSA in the conscious canines [57], which are trained to lie down quietly and sleep in the laboratory [58, 59].
8.4.2.1 Summary
Figure 8.3 shows a tracing of the electroencephalogram (EEG), ECG, respiratory variables, and electromyograms of the diaphragm (EMGdi), the transversus abdominis (EMGta) of the major expiratory muscle, and the external oblique (EMGeo) of the minor expiratory muscle during tidal breathing. A clustering of heartbeats during inspiration with the diaphragmatic contraction and a scattering during expiration with the contraction of transversus abdominis are observed.


Fig. 8.3
Polygraphic recording during spontaneous breathing in a trained canine. From top to bottom, electroencephalogram (EEG), electrocardiogram (ECG), expired CO2 concentration (%), tidal volume (liters), flow, electromyogram (EMG) of the diaphragm (EMGdi), EMG of the transversus abdominis (EMGta), and EMG of the external oblique (EMGeo) are displayed with respiratory frequency of 12 (/min) under spontaneous breathing. Respiratory sinus arrhythmia of a clustering of heartbeats during inspiration and a scattering during expiration is clearly seen with frequency of 63 (/min). Note that the dog is sleeping according to the EEG and behavioral criteria, the EMGta of the major expiratory muscle is activated and the EMGeo of the minor one is not recruited during tidal breathing
The canine sleeps spontaneously (non-REM sleep) according to the EEG and behavioral criteria [58, 60]. From the standpoint of respiratory control, the state of non-REM sleep is important, since the ventilation critically depends on metabolic control, excluding the non-metabolic (behavioral) influences on the respiratory control system [61]. Moreover, in sleeping canines, the effects of anesthetics or drug-induced levels of consciousness do not have any influence on the neural control of respiration, circulation, and their interaction [58].
8.4.2.2 Implications
In spontaneous tidal breathing, the central respiratory control modulates to “breathe in” the air by activating the EMGdi during inspiration and to “breathe out” the air by activating only the EMGta, not the EMGeo, during expiration. It is noteworthy that, only in stimulated breathing, the EMGeo of the minor expiratory muscle is recruited according to the quality and intensity of the stimulus [57]. The interacting respiratory/circulatory centers seem to generate the differential heartbeats between their clustering with the diaphragmatic contraction during the inspiratory phase and their scattering with the contraction of the transversus abdominis during the expiratory phase.
The results of this experiment have led us from the legendary concept that “the respiratory muscles drive the chest wall along the trajectory for which the work of breathing is minimal [15]” to our unique concept that “RSA is not simply the secondary product of other known reflexes but has its own physiological role to minimize the working loads of not only breathing but also circulation” [3, 16].
8.4.3 Respiratory Muscles during Hypercapnia and Hypoxia (#3 Experiment)
We summarize the author’s experiment on the respiratory muscles during hypercapnia and hypoxia in trained canines [57].
8.4.3.1 Summary
The canine’s respiration and circulation were stimulated by hyperoxic hypercapnia or normocapnic hypoxia with the rebreathing method. As the result, both hypercapnia and hypoxia substantially recruited the diaphragmatic and expiratory muscles. The expiratory muscle activities were greater during hypercapnia than during hypoxia at any comparable levels of minute volume of ventilation (expiratory shift) [62].
However, the two chemical stimuli also resulted in different tidal volume and respiratory frequency at any given minute volume of ventilation. When the EMG activities were reanalyzed as a function of tidal volume, the expiratory muscle activities were the same for a given tidal volume whether induced by hypercapnia or hypoxia, but the diaphragmatic inspiratory activity was consistently greater during hypoxia than during hypercapnia (inspiratory shift) [62].
8.4.3.2 Implications
These findings might support the concept that the stimulations by hypercapnia and hypoxia result in asymmetric activation of the inspiratory and expiratory muscles to achieve the kinematics for minimal work of breathing [15]. From the teleological viewpoint, the central regulation modulates to “breathe in” the air by recruiting the diaphragm substantially during hypoxia, and it modulates to “breathe out” the air by recruiting the expiratory muscles substantially during hypercapnia.
8.4.4 RSA during Hypercapnia and Hypoxia (#4 Experiment)
We summarize the authors’ experiments on the RSA during hypercapnia and hypoxia in the trained canines [38, 63, 64].
8.4.4.1 Summary
The canine was stimulated by hypercapnia or hypoxia as in Sect. 4.3 (#3 Experiment). The effects of both stimuli on RSA were analyzed with complex demodulation, a technique with which the frequency shifts and time-dependent changes in amplitude in the rhythmic component of the biological phenomena are assessed continuously [65].
As the result, during hypoxia, the RSA magnitude decreased (after adjusting the effects of respiratory rate and tidal volume), when heart rate, mean arterial blood pressure, respiratory rate, and tidal volume increased [63]. In contrast, during hypercapnia, the RSA magnitude increased (after adjusting the effects of respiratory rate and tidal volume) along with the increased respiratory rate and tidal volume, when the heart rate and mean arterial blood pressure did not change [38].
8.4.4.2 Implications
The above findings provide support for the concept that hypercapnia and hypoxia result in asymmetric activation not only of the respiratory muscles but also the hemodynamics and, further, the RSA [64].
From the teleological viewpoint, during hypoxia, the central regulation via the vagal nerve modulates to “breathe in” the air and subsequently to distribute the O2 from the pulmonary to systemic circulation with the maximally functioning cardiac pump with sinus tachycardia, whereas the RSA is attenuated. During hypercapnia, in contrast, it modulates to “breathe out” the air and subsequently to eliminate the CO2 from the pulmonary circulation to the open air with the modestly functioning cardiac pump with the intensified RSA, which suppresses the increase in heart rate during expiration to save the “unnecessary” heartbeats.
8.4.5 Role of Vagal Nerve on Respiratory Muscles and RSA (#5 Experiment)
We summarize the author’s experiment on the role of vagal nerve on respiratory muscles and RSA in the trained canines [57].
8.4.5.1 Summary
The effects of reversible vagal blockade on respiratory muscle activation and RSA were investigated by temporarily cooling the surfaces of bilateral exteriorized cervical vagal loops [66].
Figure 8.4 is a tracing after the transient cervical vagal blockade during spontaneous sleep in the same canine of Fig. 8.3. The pattern of EMGs is obviously changed from the spontaneous tidal breathing with a frequency of 12 (/min) to the very slow and deep breathing with a frequency of 3 (/min). Although the EMGdi during inspiration is magnified to the maximal tidal volume above 2 l, the EMGta during expiration is abolished.


Fig. 8.4
Polygraphic recording during vagal blockade in a trained canine. Displayed parameters are as in Fig. 8.3. During vagal blockade, the maximal contraction of the diaphragm with frequency of 3 (/min) and the sinus tachycardia of continuously accelerated heartbeats with frequency of 168 (/min) are seen. Note that the dog is sleeping, the EMGdi is magnified during inspiration, the contraction of the EMGta during expiration is abolished, and the RSA seen in Fig. 8.3 has disappeared
8.4.5.2 Implications
When the vagal nerve is blocked temporarily at the level of the cervical vagal trunk, both of the afferent vagal input from the lungs and the efferent vagal output to the sinus node are abolished. The classical Hering-Breuer inflation reflex mediated with the vagal nerve is eliminated [66, 67], and the disappearance in the “inspiratory-off-switch” reflex generates very slow and deep breathing. The findings of the EMGs before and after the vagal blockade suggest that the increases in the expiratory muscle activity are generated by lung inflation, which is known to augment the abdominal motor activity through an afferent vagal mechanism [57, 68].
The findings indicate that afferent vagal stimuli play an important facilitating role in the expiratory muscle activation, and hence in the chest wall movement, to achieve the minimal work of breathing by suppressing the excessiveness of lung inflation.
The findings of the ECG imply that the activity of RSA is to depend on the afferent vagal inflow from the lungs and the respiratory (phasic) modulation of vagal outflow to the sinus node. The findings also imply that the vagal nerve is to play the important roles not only in generating the RSA to achieve the minimal work of circulation, saving “unnecessary” heartbeats during expiration, but also in maintaining the homeostasis with the cardiopulmonary works to be minimal, which might serve for the wisdom of the body [13].
8.5 Clinical Significances of CSR and Entrainment with CSR
8.5.1 Clinical Significance of CSR
In an approximately 30 % of congestive heart failure patients, CSR of a cyclical fluctuation with episodes of central apneas/hypopneas alternating with episodes of hyperventilation is found [69–71], and it is more often found during nighttime sleep than daytime wakefulness [72, 73].
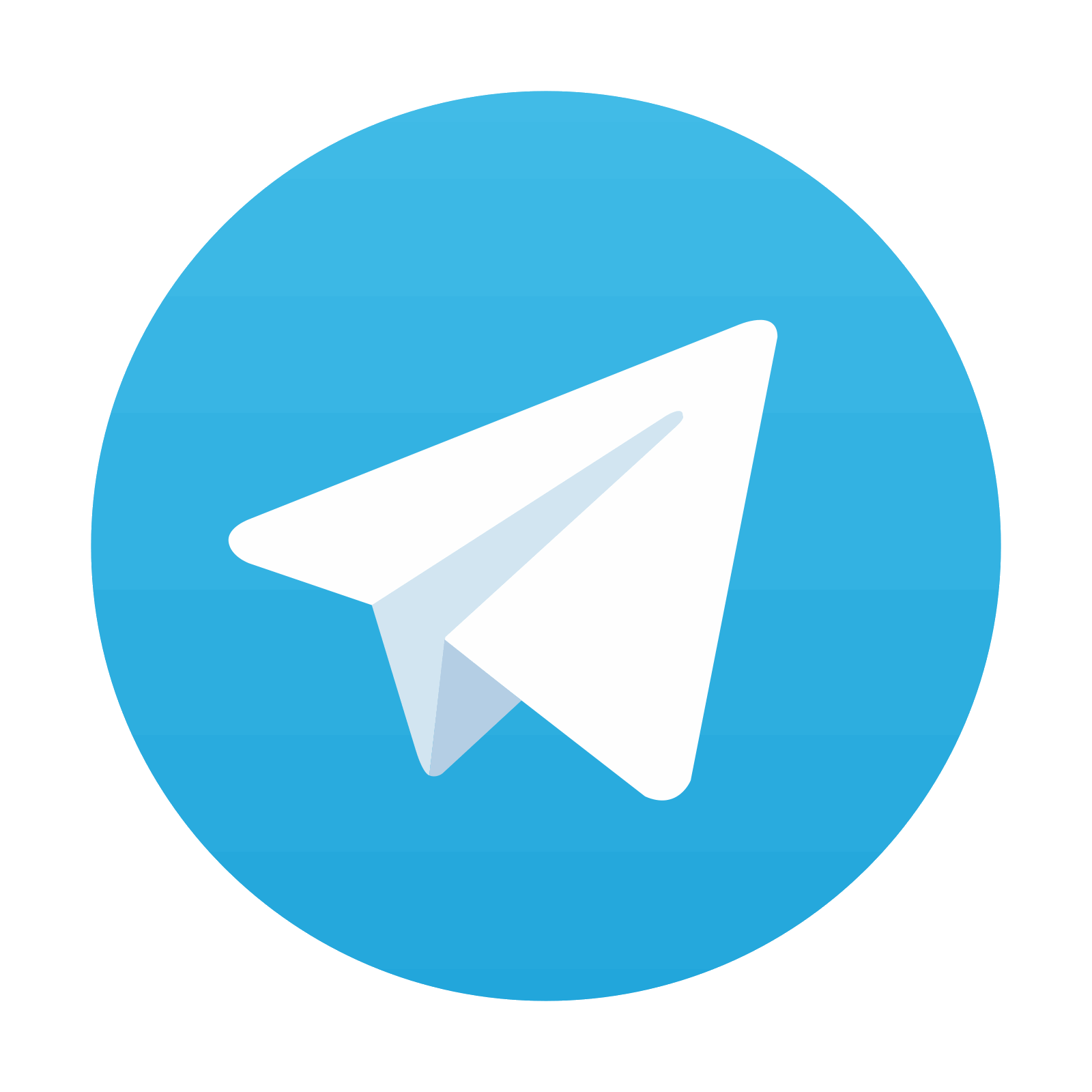
Stay updated, free articles. Join our Telegram channel
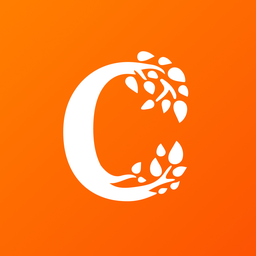
Full access? Get Clinical Tree
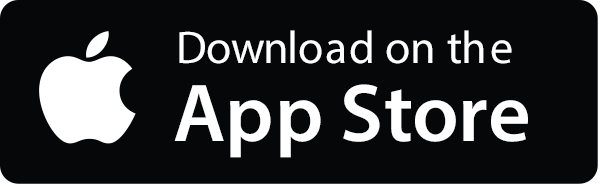
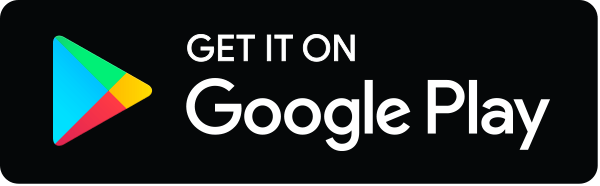