Deep brain stimulation (DBS) is an established treatment for Parkinson’s disease, and is increasingly used for other neuropsychiatric conditions including epilepsy. Nevertheless, neural mechanisms for DBS and other forms of neurostimulation remain elusive. The authors measured effects of responsive neurostimulation on intracranially recorded activity from participants in a clinical investigation to assess the safety of an implantable responsive neurostimulation system in epilepsy (RNS™ System, NeuroPace, Inc.). Neurostimulation acutely suppressed gamma frequency (35–100 Hz) phase-locking. This may represent a therapeutic mechanism by which responsive neurostimulation can suppress epileptiform activity and disconnect stimulated regions from downstream targets in epilepsy and other neuropsychiatric conditions.
Well established as a modality for the treatment of Parkinson disease, deep brain stimulation and other direct stimulations of neural tissues are increasingly being investigated to treat several neurologic and psychiatric conditions, including epilepsy (for review see ), depression, obsessive-compulsive disorder, and Tourette syndrome. Despite this increasing potential clinical utility, the mechanisms by which deep brain stimulation and other forms of neurostimulation, including electroconvulsive therapy, transcranial magnetic stimulation, and vagus nerve stimulation, modulate neuronal activity remain unknown. Deep brain stimulation and other forms of intracranial neurostimulation require mechanistic explanation at multiple levels: (1) how does neurostimulation affect directly stimulated neurons and their processes, (2) how do these direct local effects of stimulation acutely affect patterns of large-scale activity in populations of neurons, and (3) how do these effects ultimately alter macroscopic activity in brain regions over the long term. Recent work suggests that deep brain stimulation in the subthalamic nucleus may alleviate the symptoms of Parkinson disease by exciting axons from distant, possibly cortical, structures. Imaging work suggests that in depression, deep brain stimulation in axons near the subgenual cingulate (Cg25) may ultimately lead to downregulation of activity in the Cg25 and consequent changes in other interconnected regions. In this article, we focus on how intracranial neurostimulation affects synchronous rhythmic activity in populations of neurons. Disrupting synchronous activity may be an important therapeutic mechanism for deep brain stimulation in Parkinson disease and is also likely to be critically important for the treatment of epilepsy, which is, almost by definition, characterized by abnormal neural synchrony. We describe the effects of neurostimulation on rhythmic activity recorded intracranially from the neocortex and hippocampus in patients with epilepsy participating in a clinical investigation of an implantable responsive neurostimulation (RNS) system (RNS System, NeuroPace, Inc, Mountain View, CA, USA). We found that responsive stimulation acutely suppresses phase locking between gamma-frequency rhythmic activities recorded at different locations.
Methods
Electrocorticographic Recordings
Electrocorticographic (ECOG) signals were recorded from 65 patients participating in a feasibility clinical trial of the responsive stimulation System. This was a multicenter trial conducted between 2004 and 2007 designed to demonstrate adequate safety and provide preliminary evidence for effectiveness. The trial was approved by the Food and Drug Administration and investigational review boards of each center, and informed consent was obtained from all patients.
The responsive stimulation System provides responsive cortical stimulation via a cranially implanted programmable neurostimulator connected to 1 or 2 recording and stimulating depth and/or subdural cortical strip leads that are surgically placed in the brain according to the seizure focus. The neurostimulator continually senses ECOG activity and is programmed by the physician to detect abnormal ECOG activity and then provide stimulation. Forty-one subjects had leads implanted in the neocortex, 19 had leads implanted in the hippocampus, and 5 had leads implanted in the hippocampus and neocortex. In total, 95 patients had leads located in the neocortex, and 29 had leads in the hippocampus. Two channels of bipolar recordings were available for each lead. Whenever recording sites or the recording montage changed, data from that patient were treated as a new data set, resulting in 146 data sets.
The cranially implanted neurostimulator processes the signals in real time, using detection parameters that were unique to each patient and adjusted over time to detect epileptiform activity. When stimulation was enabled, detection events were followed after a short latency (typically 60–300 microseconds) by electrical stimulation (typically high frequency; >90% of stimulation occurred at frequencies between 100 and 333 Hz, delivered pulses that were 120–200 microseconds wide, and lasted 100–200 milliseconds). ECOG records (sampled at 250 Hz and typically 60–180 seconds in duration) were stored in response to preprogrammed events (such as an individual detection event or multiple consecutive detection events).
Selection of ECOG Data
Some ECOG records contained multiple detection events, each of which would be followed by stimulation if stimulation was enabled. To minimize effects from one stimulation that might affect the analysis of later stimuli, we only analyzed data corresponding to the first stimulation event in each ECOG record. The wavelet analyses described later occurred at discrete time points, which were defined relative to the detection event. In ECOG records containing responsive stimulation, these time points were always measured relative to the detection event immediately preceding the stimulation. This measurement allowed us to compare activity at corresponding time points in ECOG records that contained stimulation (after detection events) and those containing detection events only. During the period immediately following stimulation, signals could be saturated or blanked (ie, no signal available for analysis) or contain transient low-frequency (<1 Hz) artifacts. To ensure that our results were not affected by these sorts of stimulation artifacts, we verified that ECOG signals from every recording channel were nonzero and nonsaturated throughout a window surrounding each time point. This window was large enough to include all time points that might contribute to the analysis via temporal filtering and the wavelet transformation. Any ECOG records that did not meet these criteria were excluded from the analysis. We only analyzed data sets in which a minimum of 10 ECOG records with and without stimulations were available to calculate the phase-locking statistic.
Wavelet Analysis
To study rhythmic activity, we first computed the discrete wavelet transform of activity in each channel at each time point. We based our analysis on a previously described approach. Specifically, for each frequency, f , we first band-pass filtered recordings between f ± 2.5 Hz ( Fig. 1 B) and then convolved the filtered signal with a wavelet of frequency f to obtain an amplitude and a phase given by
where σ = 4/3 f and f is the frequency.

Measuring Phase Locking
Following the approach described by Lachaux and colleagues, we used phases obtained from the discrete wavelet transformation described earlier to calculate a measure of phase locking. For each pair of recordings from each data set, we computed phase differences, converted these to unit vectors in the complex plane (see Fig. 1 C), and used the amplitude of the average of these unit vectors to measure the degree of phase locking or synchrony at frequency f . We refer to this measure as the phase-locking statistic.
Determination of Statistically Significant Phase Locking
We determined whether a particular value of the phase-locking statistic was statistically significant using bootstrapping. Specifically, we computed 1000 random values of the phase-locking statistic. Each of these was the sum of N unit vectors in the complex plane, each of which had a pseudorandom phase. N is the number of phases (or unit vectors) that was used to calculate the original phase-locking statistic. Then, we obtained a P value by determining how often the actual calculated phase-locking statistic exceeded these pseudorandom values of the phase-locking statistic.
Results
We studied how responsive stimulation affected rhythmic activity in the neocortex and hippocampus. As described in the “Methods” section, ECOG signals were recorded from chronically implanted intracranial electrodes in patients with epilepsy. Briefly, a cranially implanted neurostimulator continually senses ECOG activity and detects abnormal ECOG activity. If stimulation was enabled, detection events were followed after a short latency by electrical stimulation. Brief ECOG records were stored containing detection events (with and without stimulation). Fig. 1 A shows ECOG data from 2 distinct channels recorded simultaneously during a detection event followed by stimulation.
To study rhythmic activity, we measured both the amplitude of rhythmic activity and the degree of phase locking, or synchrony, between rhythmic activities recorded simultaneously from different channels, based on a previously described approach. The detailed method for computing this measure of phase locking, referred to as the phase-locking statistic, is described in the “Methods” section. This measure of phase locking is similar to coherence, but does not require stationary signals, and measures the phase relationship independent of amplitude. We compared the strength and synchrony of oscillations 1.0, 0.5, and 0.2 seconds before detection events and 1.0, 1.2, and 2.0 seconds after these events. As described in the “Methods” section, we omitted data containing stimulation artifacts. We measured the statistical significance of phase locking by bootstrapping as described in the “Methods” section. We analyzed frequencies between 10 and 100 Hz in 5-Hz intervals, although in some cases we then grouped frequencies into 4 bands: 10 to 30 Hz representing alpha and beta bands, 35 to 50 Hz representing low-gamma band, 55 to 80 Hz representing the mid-gamma band, and 85 to 100 Hz representing the high-gamma band. Low frequencies (<10 Hz) were excluded because of the potential amplifier-sensing artifact, whereas high frequencies (>100 Hz) were excluded because recordings included a low-pass analog filter of approximately 80 to 90 Hz to minimize aliasing for signals lower than 125 Hz.
To study the effects of responsive stimulation on phase locking, we focused on those ECOG records containing statistically significant phase locking ( P <.05) either before or after detection events (with and without stimulation). The presence of phase locking was similar in ECOG records containing stimulations (16.8% of all electrode pairs exhibited phase locking) and those containing detection events that were not followed by stimulations (16.3% of all electrode pairs exhibited phase locking). This fraction was much higher than would be expected by chance (9%). To compare changes in phase locking that occur after rhythmic stimulation with those occurring after detection events in the absence of stimulation, we looked at data collected from the same patients, using the same electrode configurations, in which both the stimulation and detection-only data sets contained statistically significant phase locking at the same frequency. There were 239 such cases, and these were drawn from 60 data sets (out of 129 total data sets).
Fig. 2 shows phase locking, as a function of time relative to the detection event, for these cases (stimulation present vs detection events only). Note that the phase-locking statistic can vary between 0 (no phase locking) and 1 (perfect phase locking). Both the data containing stimulations and data containing detection events only show a sharp rise in phase locking from 500 to 200 milliseconds preceding the detection event and an eventual return to the baseline level of phase locking. However, after stimulations, the phase-locking 1 second after the detection event decreases by an average of −8% ± 4%. By contrast, in the absence of stimulation, the phase locking continues to rise, by an average of 3% ± 4%. As a result, the phase locking is significantly lower after stimulations than in the absence of stimulation ( P <.05; 2-tailed t test).

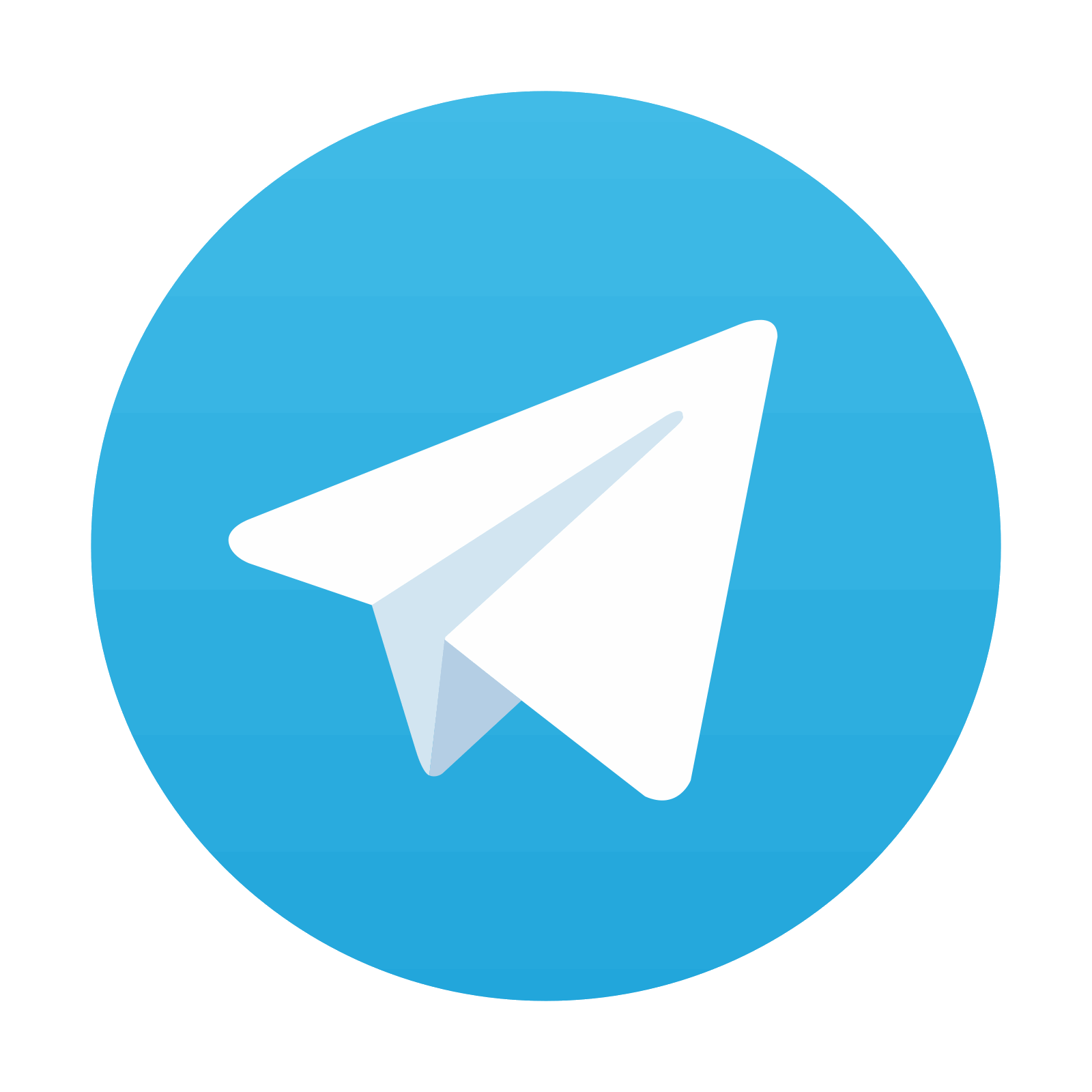
Stay updated, free articles. Join our Telegram channel
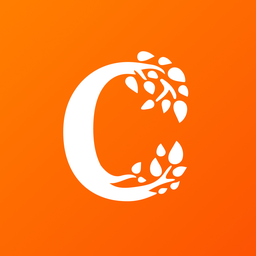
Full access? Get Clinical Tree
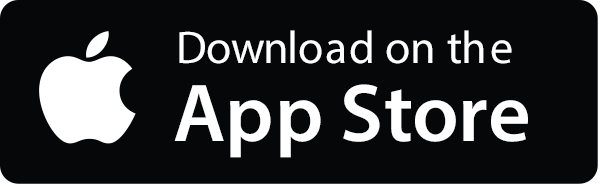
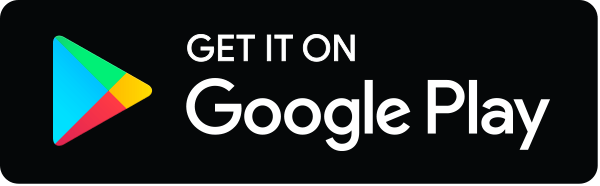
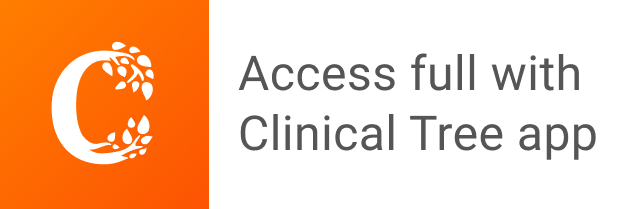