Brain metastasis is one of the most common diagnoses encountered by neurologists, neurosurgeons, radiologists, and oncologists. The aim of this article is to review imaging modalities used in the diagnosis and follow-up of brain metastases. Through the use of various imaging techniques more accurate preoperative diagnosis and more precise intraoperative planning can be made. Post-treatment evaluation can also be refined through the use of these imaging techniques.
Brain metastasis is one of the most common diagnoses encountered by neurologists, neurosurgeons, radiologists, and oncologists. The aim of this article is to review imaging modalities used in the diagnosis and follow-up of brain metastases. Through the use of various imaging techniques more accurate preoperative diagnosis and more precise intraoperative planning can be made. Post-treatment evaluation can also be refined through the use of these imaging techniques.
Metastatic disease to the brain affects approximately 10% to 20% of cancer patients but can be much higher depending on which data series is reviewed (radiologic, surgical, or autopsy). The overall incidence of metastatic brain disease is increasing, which has paralleled the rise in primary lung cancer. Another factor contributing to this increase is the longer survival rates in patients with metastases due to improvements in therapy and better radiologic detection. With respect to autopsy and clinical data, which are more difficult to verify, the incidence of brain metastases is likely to be higher than that of primary brain tumors.
The general location of metastases in the brain is consistent with blood flow, with approximately 80% in the cerebral hemispheres, 15% in the cerebellum, and 5% in the brain stem. Brain metastases occur secondary to hematogenous spread of neoplastic cells to the brain or tumor emboli. Metastases may also involve the calvarium, dura, and leptomeninges, but parenchymal metastases are the most common. The most common sources of metastases are lung (40% to 50%), breast (15% to 25%), and melanoma (5% to 20%). Gastrointestinal and renal cell carcinomas are less common but not infrequent. Melanoma is the most likely neoplasm to metastasize to the brain (approximately 50%). Although metastases may be multiple (eg, melanoma), solitary metastases are not uncommon, as seen in lung and breast cancers (approximately 50%).
General imaging characteristics
There is no particular location predilection with regard to tumor origin, with a few exceptions. Renal cancer is seen slightly more commonly infratentorially ( Fig. 1 ). This may be secondary to retrograde dissemination via Batson plexus, which may account for a slightly higher incidence of posterior fossa metastases from retroperitoneal tumors, such as those of the gastrointestinal tract, bladder, kidney, and uterus.

A common general location of parenchymal metastases is the interface of cortical brain and white matter tracts (ie, the gray-white junction), which may be accounted for by the significant change in size of arterioles from cortex to white matter, resulting in lodging of metastases in this location ( Fig. 2 ).

In addition, metastases are usually surrounded by extensive vasogenic edema. This may be due to tumor capillaries’ increased permeability with respect to normal capillaries as well as temporary occlusion as a result of neoplastic cell growth. This abnormal vascular permeability allows macromolecules to travel easily into the perivascular and then interstitial spaces. These changes cause increase in pressure on the arterial side of capillary bed and increase in transudation. As a result, peritumoral vasogenic edema, which follows white matter, interdigitating with uninvolved gray matter can be observed on CT but is more obvious on MRI. Cortical metastases, therefore, may not exhibit much surrounding edema because the cortex has a tight interstitium. These metastases may be missed without contrast on both CT and MRI, due to their lack of reactive edema.
Most metastases are hypodense on noncontrast CT but may not be well visualized amidst the surrounding hypodense edema. The pattern of MRI signal intensity of different metastases is not usually helpful in providing a specific diagnosis, but a few general observations have been made. Most metastases demonstrate prolonged relaxation time (high T2 signal). Metastases may demonstrate cystic necrosis. Low T1 and high T2 signal, however, does not confirm the cystic nature of a neoplasm. Sharp demarcation of lesion with rim enhancement should also be observed. Unlike simple cysts, cystic necrosis demonstrates high signal on fluid-attenuated inversion recovery (FLAIR) sequence (which suppresses signal intensity that is cerebral spinal fluid [CSF]-like). Usually, the T1 rim is not high as expected with an abscess. Necrosis, however, can also shorten relaxation time (low T2 signal). This may be secondary to paramagnetics within the tumor (eg, iron or copper) or free radical peroxidation.
Hemorrhage can be associated with metastatic neoplasm and is easily identified acutely on CT as hyperdensity, which decreases in attenuation after 3 to 4 days, becoming isodense and subsequently hypodense by approximately 7 to 10 days. The paramagnetic effects of blood breakdown products allow intratumoral hemorrhage to be readily identified on MRI for weeks to years after the acute event. Approximately 20% of metastases are hemorrhagic and a hemorrhagic neoplasm is more likely to be metastatic than primary. The most common hemorrhagic metastases are melanoma, small cell lung cancer, thyroid cancer, choriocarcinoma, and renal cell carcinoma Fig. 3 .

Hemorrhagic metastases, like other hemorrhagic neoplasms, may demonstrate heterogeneous intensity pattern (due to repeated bleeding), an incomplete hemosiderin rim, disproportionate amount of edema for hematoma size, or persistent/increasing edema over several weeks. These findings suggest an underlying neoplasm. Additional characteristics that may help to distinguish an uncomplicated parenchymal hematoma from a hemorrhagic metastasis include delayed evolution of hemorrhage within the neoplasm (persistent deoxyhemoglobin-usually seen only for 3 to 5 days) and T1 shortening of intracellular methemoglobin within and not at the periphery of the lesion. Identification of enhancing, non-hemorrhagic tumor component can often seal the diagnosis of hemorrhagic metastasis.
Melanin-containing melanoma metastasis also typically demonstrates T1 shortening (high signal on T1) and isointense T2 signal due to free radical content of melanin, unlike intracellular methemoglobin, which demonstrates high T1 and very low T2 signal. Confounding this observation is the occurrence of hemorrhage within melanoma metastases. With associated use of a susceptibility sequence, T2*, further improved detection of melanoma metastases is possible. At times, melanoma lesions are detected only on T2*-weighted (also known as gradient-echo) sequence ( Fig. 4 ). Amelanotic melanoma demonstrates T1 hypointensity and T2 hyperintensity.

Hypervascularity can be associated with a metastatic lesion, with possible subsequent hemorrhage. Prominent flow voids seen on T2-weighted sequence represent enlarged vessels. The differential diagnosis includes hemangioblastoma, glioblastoma multiforme (GBM), anaplastic oligodendroglioma, and metastatic renal cell carcinoma. Gradient recalled echo (GRE) sequence can confirm both the presence of hemorrhage and prominent vessels.
Low T2 signal (similar to gray matter) is seen in some metastatic neoplasms and may offer a clue to tissue of origin. Mucin-secreting tumors (gastrointestinal and genitourinary adenocarcinomas and at times lung) and hypercellular neoplasms (high nuclear-to-cytoplasmic ratio, small round cell tumors), such as lymphoma (both primary and metastatic), medulloblastoma/primitive neuroectodermal tumors, pineoblastoma, and neuroblastomas, often demonstrate low T2 signal. These lesions also demonstrate slightly restricted diffusion (high signal on diffusion weighted imaging [DWI] with corresponding reduced apparent diffusion coefficient [ADC] maps), again due to their high cellularity and decreased interstitial space and water Fig. 5 . Small cell lung cancer, amelanotic melanoma, and some hemorrhagic metastases may also have low T2 signal.

Calcified metastases are rare and most commonly due to ovarian cancer and osteosarcoma metastases. Calcifications are best seen on CT, because MRI may not provide sufficient discrimination between calcification and hemorrhage.
Contrast-enhanced MRI and CT
Contrast enhancement is necessary to evaluate for metastatic disease, because nearly all metastases demonstrate enhancement due to lack of blood-brain barrier and lack of blood-tumor barrier in their vascular endothelium. The pattern of enhancement can vary significantly among different metastatic neoplasms but again offers little clue as to the tissue origin. The enhancement pattern may be solid, peripheral, or nodular. The wall characteristics may help distinguish a neoplasm, either metastatic or primary, from a benign process, such as abscess or demyelinating disorder. Irregular thick or nodular enhancement is more commonly seen with malignant neoplasm than with benign conditions, which usually produce smooth thin wall enhancement.
MRI without and with contrast is the imaging modality of choice in evaluating patients with suspected metastases. Head CT is often performed at the initial screening or in an emergency setting to exclude hemorrhage. MRI, however, is superior to CT either without or with contrast in the detection and evaluation of metastases. Approximately 19% of patients with a solitary metastasis on contrast-enhanced CT have multiple metastases on a contrast-enhanced MRI. Improved detection with postcontrast MRI is secondary to superior soft tissue contrast, lack of bone artifact, decreased partial volume averaging, and better enhancement with paramagnetic MRI agents (gadolinium agents) versus CT iodinated contrasts. In addition, MRI is better able to identify posterior fossa/infratentorial lesions and leptomeningeal metastatic disease.
Identification of lesions on MRI depends primarily on size of lesion and degree of contrast of lesion to background. The timing and dosing of imaging after gadolinium contrast administration play a major role in visualizing metastases. Delayed imaging postcontrast administration may help in detection of lesions that are 5 mm or smaller. Waiting 5 to 35 minutes with standard dose improves detection. Higher dosing also detects more lesions and approximately 10% of such cases alter management. With triple dosing, Yuh and colleagues found improved detection of multiple metastases. Delineation of these lesions may be important in developing a therapeutic strategy for a patient. Furthermore, prognostic factors after treatment vary depending on the number of cerebral metastases.
Another option for improved visualization of smaller metastases is the use of higher-field MRI scanners. 3T MRI scanners, now readily available in clinical practice, have higher signal-to-noise ratio and longer T1 relaxation time of gray and white matter, which results in more shortening of T1 relaxation time by the uptake of contrast. Flow artifacts are more noticeable at higher field strengths and can mimic small metastases but can be readily reduced with saturation pulses.
MR techniques, such as magnetization transfer with single-dose gadolinium diethylenetriamine penta-acetic acid (Gd-DTPA), have also been found as efficient as triple-dose Gd-DTPA. Magnetization transfer allows better visualization of intracranial lesions by preapplying an off-resonance radio frequency pulse, which suppresses the signal of the background tissue. Knauth and colleagues studied 24 patients with 34 enhancing intracranial lesions. No difference was found in number of lesions detected when comparing standard-dose magnetization transfer T1-weighted images with triple-dose T1-weighted images. Triple-dose magnetization transfer T1-weighted studies further increase lesion-to-white matter contrast but do not show additional lesions.
Use of magnetization transfer technique and higher-field magnet scanners, rather than triple dosing, should be considered, particularly in the setting of patients with renal disease, given recent concerns for nephrogenic systemic fibrosis or nephrogenic fibrosing dermopathy, which has been seen in patients with moderate to severe end-stage renal disease after the administration of a high dose of a gadolinium-based contrast agent.
Contrast-enhanced MRI and CT
Contrast enhancement is necessary to evaluate for metastatic disease, because nearly all metastases demonstrate enhancement due to lack of blood-brain barrier and lack of blood-tumor barrier in their vascular endothelium. The pattern of enhancement can vary significantly among different metastatic neoplasms but again offers little clue as to the tissue origin. The enhancement pattern may be solid, peripheral, or nodular. The wall characteristics may help distinguish a neoplasm, either metastatic or primary, from a benign process, such as abscess or demyelinating disorder. Irregular thick or nodular enhancement is more commonly seen with malignant neoplasm than with benign conditions, which usually produce smooth thin wall enhancement.
MRI without and with contrast is the imaging modality of choice in evaluating patients with suspected metastases. Head CT is often performed at the initial screening or in an emergency setting to exclude hemorrhage. MRI, however, is superior to CT either without or with contrast in the detection and evaluation of metastases. Approximately 19% of patients with a solitary metastasis on contrast-enhanced CT have multiple metastases on a contrast-enhanced MRI. Improved detection with postcontrast MRI is secondary to superior soft tissue contrast, lack of bone artifact, decreased partial volume averaging, and better enhancement with paramagnetic MRI agents (gadolinium agents) versus CT iodinated contrasts. In addition, MRI is better able to identify posterior fossa/infratentorial lesions and leptomeningeal metastatic disease.
Identification of lesions on MRI depends primarily on size of lesion and degree of contrast of lesion to background. The timing and dosing of imaging after gadolinium contrast administration play a major role in visualizing metastases. Delayed imaging postcontrast administration may help in detection of lesions that are 5 mm or smaller. Waiting 5 to 35 minutes with standard dose improves detection. Higher dosing also detects more lesions and approximately 10% of such cases alter management. With triple dosing, Yuh and colleagues found improved detection of multiple metastases. Delineation of these lesions may be important in developing a therapeutic strategy for a patient. Furthermore, prognostic factors after treatment vary depending on the number of cerebral metastases.
Another option for improved visualization of smaller metastases is the use of higher-field MRI scanners. 3T MRI scanners, now readily available in clinical practice, have higher signal-to-noise ratio and longer T1 relaxation time of gray and white matter, which results in more shortening of T1 relaxation time by the uptake of contrast. Flow artifacts are more noticeable at higher field strengths and can mimic small metastases but can be readily reduced with saturation pulses.
MR techniques, such as magnetization transfer with single-dose gadolinium diethylenetriamine penta-acetic acid (Gd-DTPA), have also been found as efficient as triple-dose Gd-DTPA. Magnetization transfer allows better visualization of intracranial lesions by preapplying an off-resonance radio frequency pulse, which suppresses the signal of the background tissue. Knauth and colleagues studied 24 patients with 34 enhancing intracranial lesions. No difference was found in number of lesions detected when comparing standard-dose magnetization transfer T1-weighted images with triple-dose T1-weighted images. Triple-dose magnetization transfer T1-weighted studies further increase lesion-to-white matter contrast but do not show additional lesions.
Use of magnetization transfer technique and higher-field magnet scanners, rather than triple dosing, should be considered, particularly in the setting of patients with renal disease, given recent concerns for nephrogenic systemic fibrosis or nephrogenic fibrosing dermopathy, which has been seen in patients with moderate to severe end-stage renal disease after the administration of a high dose of a gadolinium-based contrast agent.
DWI
DWI is commonly used in MRI brain studies. DWI reflects the microscopic motion of water in tissue is usually disorganized or Brownian motion. DWI depends primarily on water motion in the extracellular space, with increased signal seen when it is not moving freely. To quantify the degree of water motion, ADC maps are needed, which depict true restriction as dark. The more restricted the motion of water, the lower the ADC value, with darker ADC maps. Conventional DWI imaging is commonly used in the setting of acute stroke where decreased diffusion is present, thought to be secondary to cytotoxic edema and decreased extracellular space.
Necrotic neoplasms can be differentiated from bacterial abscess using DWI and ADC maps. Bacterial abscesses tend to demonstrate increased DWI signal and corresponding decreased ADC values. This reduced diffusion may be due to decreased movement of water molecules by bacteria, inflammatory cells, cellular debris, and macromolecules, such as fibrinogen, found in viscous purulent fluid. In addition, bacterial abscesses tend to demonstrate a complete or partial, markedly hypointense T2 rim. This finding, together with restricted diffusion, can accurately discriminate cystic or necrotic neoplasm from brain abscess ( Fig. 6 ). In partly treated abscesses, however, restricted diffusion may not be observed.
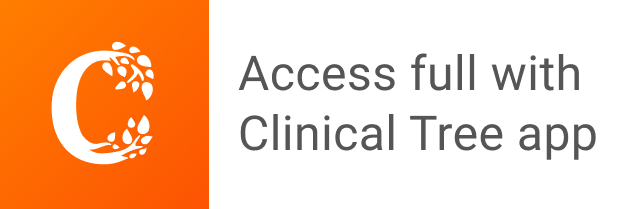