Fig. 1
Neurobiological processes that facilitate the consolidation of memory . A learning experience simultaneously activates time-sensitive storage systems in several brain regions and, particularly if the experience is an emotionally arousing one, activates the release of stress hormones from the adrenal gland and norepinephrine in the basolateral amygdala. The specific brain regions activated are dependent on memory type, and the activity in the amygdala modulates consolidation by affecting the plasticity in other regions (from McGaugh 2000)
Also important to our discussion are the various stages of memory processing. Encoding represents the first stage of the process and encompasses the initial acquisition of new information, which is transformed into mental representations in the brain. The consolidation stage then stabilizes these neural representations over subsequent hours, days, and years, increasing resistance to interference from other competing information. This crucial stage is still not fully understood, with suggestions that it be broken down into substages of stabilization and enhancement (Walker and Stickgold 2006) or that it goes beyond simple stabilization and enhancement to produce qualitative changes in memory representations (for review, see Payne and Kensinger 2010; Payne 2011). The third and final stage is retrieval of the stored memory . Although sleep facilitates the encoding of new memories (Yoo et al. 2007; Van Der Werf et al. 2009) and may smooth the path to effective retrieval, the majority of studies on sleep and declarative memory focus on consolidation, as we do below.
3 Neurochemical and Electrophysiological Properties of Sleep Stages and Their Relationship to Memory Processes
To fully appreciate the body of literature examining the role of sleep in declarative and emotional memory consolidation , it is important to first discuss the properties of sleep itself. Sleep is not a unified, constant state or a period of inactivity. Rather, it is a dynamic process that progresses through many stages, each with unique neurochemical and electrophysiological properties. As covered in Chap. 1 (Deboer, 2013), sleep is generally delineated as non-rapid eye movement (NREM) sleep and rapid eye movement (REM) sleep . NREM sleep is comprised of stages 1–4 as defined by Rechtschaffen and Kales (1968), or more recently redefined by the American Academy of Sleep Medicine (2007) as N1–N3, with each stage defined by specific frequency ranges (see Chap. 1). Collectively, Stages 3 and 4 (or N3) is known as slow-wave sleep (SWS) and represents the deepest sleep state. In a typical night of sleep , the brain progresses through all the stages before beginning the sequence again, repeating this cycle about every 90 min throughout the night. However, the distribution of these stages across a typical night of sleep is not entirely even. The first half of the night contains a majority of the night’s SWS, whereas the amount of REM in the second half of the night is nearly doubled from the first (Fig. 2; Payne 2011).
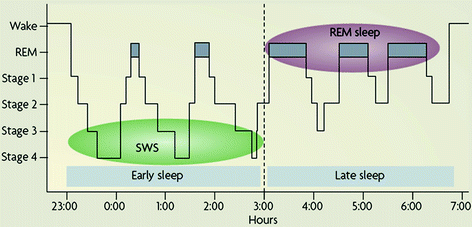
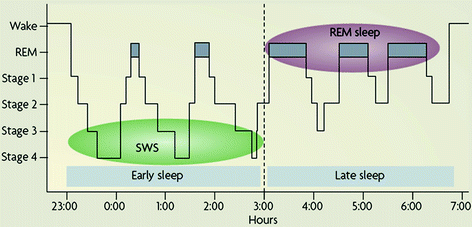
Fig. 2
Slow wave sleep dominates the first half of the night, but during the latter half, REM sleep dominates (from Payne 2011)
Stage 2, SWS, and REM sleep are most relevant to our discussion on declarative and emotional memory within this chapter, so an understanding of the neurochemical and electrophysiological dynamics within these stages is in order as it serves as the foundation for prominent theories regarding memory processing during sleep (see Sect. 4).
3.1 Non-rapid Eye Movement Stage 2 Sleep
Upon falling asleep, the brain shifts from the waking state, through the Stage 1 transitory state, into Stage 2 sleep . This stage is marked by sleep spindles, high-frequency bursts in the sigma band (12–15 Hz), and K-complexes, brief high-amplitude, negative, high-voltage peaks of approximately 100 µV followed by a slower positive complex and a final negative peak, on background brain activity in the 5–8 Hz range. Aminergic tone (norepinephrine, NE; serotonin, 5-HT) as well as cholinergic tone (acetylcholine, ACh) are similar to that seen in SWS, both at relatively low levels compared to a waking state (Stickgold et al. 2001; Smith et al. 2004 for review).
Stage 2 sleep spindles can differ in frequency and regions of generation, from slow spindles in the 12–14 Hz range concentrated over frontal locations to fast spindles (14–16 Hz) originating in centro-parietal loci (Sterman et al. 1978; Tanguay et al. 1975; Zeitlhofer et al. 2003). Sleep spindles have been proposed to play a role in reactivating a memory for further synaptic modifications (Rosanova and Ulrich 2005) and implicated in assimilating new and old information (Tamminen et al. 2010). Comparison of baseline spindle activity (quantity and density) to activity following intensive learning has revealed changes that are positively correlated with memory performance on different types of memory tasks, including declarative memory (Gais et al. 2002; Clemens et al. 2005; Schabus et al. 2004).
3.2 Slow-Wave Sleep
As the brain moves into deep NREM sleep or SWS, activity is characterized by high-amplitude, low-frequency oscillations, or delta waves (0.5–4 Hz). Also present are sharp-wave ripples, or fast hippocampal neural oscillations (150–250 Hz), which are grouped by and occur in the transition between the upstates of the slow oscillations (Steriade et al. 1993; De Gennaro and Ferrara 2003; Battaglia et al. 2004; Mölle et al. 2006). These ripples are temporally correlated with sleep spindles (Siapas and Wilson 1998; Sirota et al. 2003) and are a key component in a major theory regarding memory consolidation (Buzsaki et al. 1983; Buzsaki 1989, see Sect. 4). Positron emission tomography (PET) studies have investigated regional cerebral blood flow (rCBF) during SWS and found an overall deactivation of the brain compared to wakefulness, including areas of the midbrain, limbic areas, and higher cortical areas such as the dorsolateral prefrontal cortex (DLPFC; Braun et al. 1997).
Additionally, noradrenergic, serotonergic, and cholinergic connections in the cortex are at low firing levels, with levels of ACh at their very lowest during SWS (less than one-third of that seen during active wake; see Fig. 3). We emphasize the role of ACh and NE throughout our description of the sleep stages because these chemicals have been shown to directly affect hippocampal neurons and impact declarative memory traces. High ACh levels benefit the encoding process (during wakefulness) because this neurotransmitter suppresses glutamatergic receptors in the hippocampus, reducing connectivity between the hippocampus and long-term memory stores in the neocortex. This in turn prevents the concurrent reactivation of older, competing memories in the hippocampus and thus minimizes interference. However, reduced ACh levels during SWS facilitate feedback from the hippocampus to the neocortex by releasing most glutamatergic synapses from suppression, thus aiding declarative long-term memory processing and systems consolidation (Hounsgaard 1978; Valentino and Dingledine 1981; Rovira et al. 1983; Hasselmo and Bower 1992; Herreras et al. 1988; Hasselmo 1999; Hasselmo and McGaughy 2004). When levels of ACh are experimentally increased during SWS-rich sleep early in the night, performance on a word associates task, which typically improves following SWS, is weakened (Gais and Born 2004).
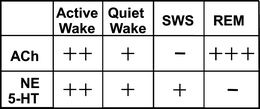
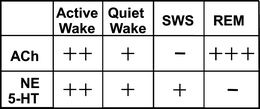
Fig. 3
Graphical representation of varying concentrations of neuromodulators across a variety of brain states. ACh Acetylcholine, NE Norepinephrine, 5-HT Serotonin
Norepinephrine released from the locus coeruleus (LC) during NREM sleep modulates activity in the neocortex and the hippocampus as well (Aston-Jones 2004). Bursts of activity from the LC correlate with sleep spindles and slow oscillations during SWS and may be implicated in memory consolidation (Gais et al. 2011). LC and NE levels have been shown to transiently increase after a period of learning (Eschenko and Sara 2008; Sara 2009). Furthermore, blocking NE activity during NREM sleep with clonidine, but not during wake, results in a reduction in sleep-dependent facilitation of memory (odor recognition), while an NE reuptake inhibitor increased memory consolidation (Gais et al. 2011).
3.3 Rapid Eye Movement Sleep
While the literature heavily implicates SWS as the most critical stage for declarative memory consolidation , there is a growing body of evidence suggesting that REM sleep may selectively facilitate emotional declarative memories (see Sect. 8). As the brain shifts into REM sleep, characterized by a drastic reduction in muscle tone and low-amplitude, high-frequency EEG patterns (tonic events of REM sleep), as well as periodic rapid eye movements (REMs) and muscles twitches (the phasic events of REM sleep) (Carskadon and Dement 1989), levels of brain activation are generally comparable or even greater than that seen during wakefulness, especially in the brainstem, basal ganglia, hippocampus, nearby limbic areas, and some cortical areas. Specifically, increased activity between amygdala, hippocampus, and cortical areas such as the mPFC (Braun et al. 1997), which are important emotional centers of the brain, points to REM as a possible ideal stage for emotional memory consolidation (Maquet et al. 1996). Further, noradrenergic connections in the cortex are virtually silent and ACh levels are about 60 % lower than in the waking state (though, see Vazquez and Baghdoyan 2001). However, in the hippocampus and surrounding regions, ACh levels are elevated above those seen during waking (Hasselmo 1999). The resulting suppression of glutamatergic receptors may again shut down the flow of information from the hippocampus to the neocortex (Hasselmo 1999), perhaps facilitating cortico–cortico interactions (Payne et al. 2004; Payne 2011). ACh elevations have also been associated with the promotion of emotional memory during REM sleep , particularly given that ACh activation in the amygdala is associated with memory enhancing effects (McGaugh 2004).
4 Theories of Sleep and Declarative Memory Processing
To fully appreciate the research that will be discussed below, we first outline the basic theories used to motivate experiments and interpret results. Sleep and declarative memory research is generally understood within two theoretical frameworks, which are based upon the neurochemical and electrophysiological properties of SWS. According to the standard two-stage theory of consolidation (Buzsaki et al. 1983; Buzsaki 1989; McClelland et al. 1995), the first stage begins with rapid encoding of new information through waking interaction with the environment. Experience initiates a flow of activation from sensory receptors, through thalamic weigh stations (with the exception of olfactory processing) to the entorhinal cortex and input layers of the CA3 region of the hippocampus, both directly and indirectly through the dentate gyrus (Chrobak and Buzsaki 1994). As described above, the high levels of ACh during the waking state facilitate this process. Subsequently, during SWS, sharp-wave ripples participate in the reactivation of neuronal networks that were most recently fired during waking, namely those representing declarative information (Pavlides and Winson 1989; Wilson and McNaughton 1994; Skaggs and McNaughton 1996; Kudrimoti et al. 1999; Nadasdy et al. 1999; Maquet et al. 2000; Louie and Wilson 2001; Hoffman and McNaughton 2002; Ji and Wilson 2007). Best demonstrated in an animal study by Wilson and McNaughton (1994), reactivation of waking neuronal activity was found to occur primarily during SWS. They examined neuronal firing patterns of place cells in the hippocampi of rats exploring an environment prior to sleep and then observed a “replay” of this activity in a time-compressed manner during SWS (however, see Hennevin et al. 2007, for a critique). This replay is thought to lead to long-term potentiation (LTP), the predominant candidate as a mechanistic explanation for synaptic consolidation within a network (Sirota et al. 2003; Brehens et al. 2005; Whitlock et al. 2006; Diba and Buzsaki 2007).
LTP is initiated by the tetanic stimulation of one neuron (the presynaptic neuron), which binds glutamate to the alpha-amino-3-hydroxy-5-methyl-4-isoxazolepropionic acid (AMPA) subtype receptors of the postsynaptic neuron and causing depolarization. Prolonged depolarization causes the magnesium block in the N-methyl-D-aspartic (NMDA) subtype glutamate receptors to be removed, allowing calcium influx and beginning a second messenger cascade resulting in activated kinases, gene transcription, and protein synthesis (Sweatt 1999; LeDoux 2002). The result of this process is an increase in surface AMPA and NMDA receptors at the synapses involved in firing the cell, dendritic growth, increased channel conductance in the postsynaptic cell, and growth of new terminal branches and synapses in the presynaptic cell. All of these changes due to LTP strengthen the connection between the two cells, making them more likely to fire together in the future (Bliss and Lomo 1973). Essentially, when a presynaptic cell takes part in firing a postsynaptic cell, they both benefit from the processes described above, becoming “wired together,” increasing the chances they will fire together again in subsequent activations, similar to the reactivation seen during SWS (Hebb 1949). The strength of the bursts of sharp-wave ripples during SWS is sufficient to induce LTP within the network (Buzsaki 1989) and some evidence ascribes a causal role to sharp-wave ripples in memory consolidation (O’Neill et al. 2010). Selective suppression of sharp-wave ripples in rats previously trained on a spatial task resulted in significant performance impairments during retesting, although it has not been determined whether the absence of ripples or disruption of neuronal replay during SWS is the exact cause of the impairment (Girardeau et al. 2009; Ego-Stengel and Wilson 2010).
An alternate theory proposed by Tononi and Cirelli (2003, 2006) ascribes an indirect role of SWS in memory processing. This theory is based on the observation that synapses are continuously potentiated through the waking hours, mostly with everyday activities but specifically with focused learning, thus depleting synaptic resources and increasing the threshold for neuronal firing. Coupled with this observation is a homeostatic increase in the need for SWS, so that as the need increases, more SWS is obtained at sleep onset, gradually decreasing over the sleeping period. According to this theory, SWS serves to globally downscale, or reduce synaptic weights, bringing them back to baseline levels so that plasticity and thus the ability to take on new information is restored. This downscaling is a result of the alternating synchronous hyper- and depolarization that occurs in cortical circuits during SWS, with the changes in membrane potential serving to reset the synaptic strengths. Regionally specific or “local” increases in slow-wave activity have been shown to correlate with the location of activation during intensive learning, so that SWS can locally and globally return the brain to baseline (Huber et al. 2004). According to this theory, memory is therefore a by-product of the active downscaling that occurs during SWS. Although there is global downscaling of synaptic strength, the synapses involved in a learned memory trace are stronger than those involved in firing during everyday waking experience. As the latter falls below threshold for firing, the memory trace stands out in an increase of the signal to noise ratio. However, it is unclear whether downscaling benefits memory by occurring in the hippocampus proper, which has conflictingly been shown to maintain firing rate over time (Buzsaki et al. 2002), or only the cortical areas potentiated by learning and associated with the memory trace.
These two theories regarding the role of SWS in declarative memory processing are not mutually exclusive, as both processes could occur simultaneously, with consolidation from the hippocampus to the neocortex happening in conjunction with global downscaling to reset the brain. Keeping these theories in mind, we will next detail the research demonstrating superior memory and refined neural network activity reflecting systems consolidation after overnight sleep and daytime naps (Sects. 5 and 6), and the deleterious effects of sleep deprivation (Sect. 7) on memory for both neutral and emotionally salient information (Sect. 8).
5 Overnight Sleep Benefits Declarative Memory
5.1 Roles for Sleep in Memory Consolidation
While it has been established that sleep promotes the consolidation of newly acquired memories, the true nature of the function of sleep in such processing continues to be disputed. Three possible roles (passive, permission, and active) have been suggested to describe the impact of sleep on memory consolidation (Ellenbogen et al. 2006), each with different proponents. The first role has been deemed the passive theory, which, as demonstrated by the conclusions drawn from early studies such as those conducted by Heine (1914) and Jenkins and Dallenbach (1924), posits that memories benefit from a period of sleep simply due to shielding from “the interference, inhibition, or obliteration of the old by the new” (Jenkins and Dallenbach 1924, p. 612). Essentially, this theory contends that sleep merely offers protection from the influence of new incoming information and thus protects recently learned information from the effects of interference for the duration of sleep (Wixted 2004; Vertes and Siegel 2005), with the memory once again susceptible to interference upon awakening.
The permissive hypothesis (Ellenbogen et al. 2006) similarly claims that sleep shields new memories by offering an opportunity for reduced interference, but it further asserts that this opportunity is only valuable if it occurs within a certain time frame following learning. Consolidation occurs during both sleep and wakefulness; however, sleep offers greater consolidation efficiency because of the reduction of interference during this state. Importantly, this effect of sleep is time sensitive, such that sleep must occur during a period in which the memory is still labile, prior to the stabilizing effects of gene expression and synaptic modifications, in order to have any consolidation benefit.
Conversely, the active hypothesis (Ellenbogen et al. 2006) posits that sleep actively facilitates memory consolidation , rather than simply protects newly learned information from interference. Unlike the previous two hypotheses, this theory stresses the importance of the unique neurochemical environment and physiological events of sleep , such as sharp-wave ripples and sleep spindles discussed earlier (see Sect. 3), for the successful consolidation of memory . A growing body of evidence supports such an active role, with research revealing changes in hippocampal activation and reorganization of brain circuitry after sleep , as well as changes in spindle density and slow-wave activity following learning. For the purposes of this chapter, the active hypothesis is most widely represented, with most discussions and reviews reflecting support for the claims of this premise.
5.2 Behavioral Evidence for an Active Role of Sleep in Memory Formation
Early support for the active hypothesis examined the contribution of particular stages of sleep over wakefulness in later declarative memory performance. Using a word-pair learning paradigm, one such study manipulated which portion of the sleep period, or an equivalent period of wake, occurred during a consolidation delay following learning (Plihal and Born 1997). Given the cyclic nature of sleep and the varying distributions of SWS and REM across the night, requiring participants to memorize word pairs prior to bedtime and sleep only for the first half of the night prior to testing ensured that the intervening sleep contained mostly SWS. Alternatively, requiring participants to sleep the first half of the night prior to learning and sleep again for the second half of the night before being awakened and tested ensured that more REM was obtained during the intervening sleep . Results confirmed that participants in the early-sleep group obtained more SWS than the late-sleep group, while the late-sleep group obtained more REM than the early-sleep group, with equivalent amounts of Stages 1 and 2 obtained between the groups. Crucially, a period of sleep improved recall of previously learned word pairs above wakefulness, but only when sleep occurred over the first half of the night, or the “early”-sleep opportunity that was rich in SWS. Because this study indicated a role for this specific stage, rather than a period of sleep in general, it strongly suggested that sleep actively promoted learning instead of simply protecting newly learned information from interference.
This “early-/late”-sleep paradigm, while not an ideal design to address potential circadian confounds, has been subsequently used to implicate active sleep processes in other types of declarative memory consolidation and modulation of physiological reactivity as well. Like episodic memories for pairs of words, spatial memories also rely on functioning of the hippocampus. This type of memory includes mental rotation tasks, such as those that require remembering placements of objects in an imaginary scene after it has been rotated from the original viewing angle (Plihal and Born 1999b). After early sleep, locating object placements in this task is more accurate than after late sleep or wake, again indicating a role for SWS. Aside from the implication of active sleep-based learning processes, investigations such as these were pivotal to establishing an association between SWS and declarative learning tasks. Although such a relationship may be oversimplified, it has survived more stringent physiological testing for certain memory types.
5.3 Physiological Evidence for an Active Role of Sleep in Memory Formation
Physiological evidence has also offered support for an active role for sleep in memory consolidation . Orban et al. (2006) demonstrated, using fMRI, increased striatal activity and changes in functional connectivity between the striatum and hippocampus, positively correlated with performance on a virtual navigation task after regular sleep versus sleep deprivation (discussed further in Sect. 7.3). The authors conclude that the covert shift in activity from hippocampus-dependent activation to striatal control reflects sleep-dependent consolidation processes. Similarly, Payne and Kensinger (2011) demonstrated a more consolidated pattern of activity for emotional memory after a night of sleep versus daytime wakefulness (discussed further in Sect. 8.1). Physiological evidence also demonstrates a SWS-declarative memory connection. Learning a virtual navigation task has been shown to increase activation, via cerebral regional blood flow (rCBF), of the right hippocampus and parahippocampal region during subsequent NREM sleep, primarily during SWS. This increase was also associated with increased performance on the task following sleep (Peigneux et al. 2004). Importantly, those who did not learn this task prior to sleep did not display the same neural activity increases in these regions, indicating that this change in brain physiology during SWS possibly resulted from the processing of this learned information. Further, reintroducing cues from a learning episode, such as an odor originally presented during the encoding of an object–location pairing task, during subsequent SWS has been shown to increase hippocampal activity during this stage, as well as lead to improved post-sleep memory for the learned material; presenting the odor during REM sleep or wake showed no such benefit (Rasch et al. 2007). Research designed to manipulate slow waves directly, increasing slow-wave activity through transcranial direct current stimulation (Marshall et al. 2004, 2006) or suppressing them with auditory tones (Van Der Werf et al. 2009), respectively, improves and impairs performance on declarative memory tasks. Finally, several studies have demonstrated increased hippocampal sharp-wave ripples during SWS after a period of learning, which were correlated with successful subsequent retrieval (Eschenko et al. 2008; Ramadan et al. 2009). Such physiological evidence compliments previous behavioral studies to support the hypothesis of active processing occurring during sleep and further suggests a SWS-specific mechanism involving activations of the hippocampus to promote declarative learning.
Several studies have demonstrated functional changes in Stage 2 sleep parameters that potentially implicate this stage in declarative memory processing as well, adding support to an active role for NREM sleep in processing of neutral declarative memories (Gais et al. 2002; Clemens et al. 2005; Schabus et al. 2004). In these experiments, subjects’ average, normal sleep spindle density (i.e., the number of 12–16 Hz bursts of activity within Stage 2 sleep per 30 s epoch), measured either during baseline sleep (Clemens et al. 2005) or during sleep following a non-learning control task (Gais et al. 2002; Schabus et al. 2004), was found to significantly increase during sleep directly following a period of intensive learning. Only those participants who showed an increase in spindle density during post-learning sleep demonstrate improved memory performance (Schabus et al. 2004). Sleep spindles have similarly been found to increase during SWS after an intensive learning session (Eschenko et al. 2006). As mentioned earlier, spindles are temporally correlated with sharp-wave ripples (Siapas and Wilson 1998; Sirota et al. 2003) as well as slow oscillations (Steriade and Timofeev 2003; Mölle et al. 2009) and can be considered plastic events themselves (Rosanova and Ulrich 2005). Therefore, it is not surprising that they play a role in declarative memory processing.
5.4 Memory After Extended Consolidation Delay Designs
Despite such mounting evidence, some continue to dispute the active hypothesis due to the unequal amounts of time that participants in sleep and wake conditions are subjected to waking interference. This is particularly problematic in designs where performance after an 8- to 12-h delay of daytime wakefulness (where subjects are continually exposed to everyday interfering input) is compared to an 8- to 12-h delay of nocturnal sleep (where a majority of the delay is spent asleep and thus provides protection from interfering stimuli; e.g., Payne et al. 2009; Wagner et al. 2004). To address this issue, recent studies have utilized extended consolidation delays (i.e., 24 h) that allow all participants to receive equal amounts of wakefulness and sleep, with only the timing of sleep varying between groups. As an example, Payne et al. (2012a) tested memory for pairs of semantically related or unrelated words following four different delay intervals. As in previous studies (Payne et al. 2009; Wagner et al. 2004), a 12-h delay following training in the morning or evening allowed for a period of daytime wake or nocturnal sleep during the consolidation period. However, a 24-h delay allowed either a period of wake followed by sleep (wake-first group) or a period of sleep followed by wake (sleep-first group). While both 12-h groups retained an equal number of the related word pairs, those receiving a period of sleep during this delay had better memory for the unrelated word pairs than those who remained awake. This finding alone provides initial evidence against an interference account, as memory for both related and unrelated word pairs would have been impacted similarly by sleep if its effect was to generally shield information from everyday normal waking interference, as predicted by the passive theory.
Further, comparison to the 24-h groups revealed that forgetting of these word pairs was doubled over the waking period when wake came prior to sleep as opposed to following sleep, while forgetting over the sleep period remained the same regardless of its placement in the 24-h delay. Similarly, Talamini et al. (2008) found that sleep not only protected memory for face–location pairings over wakefulness, but also reduced forgetting occurring during the waking portion of a 24-h delay when sleep occurred prior to wakefulness as opposed to following it (see Fig. 4). Given an interference account, the amount forgotten over the portion of the delay filled with wakefulness in these two studies would be the same regardless of its position to sleep. The fact that forgetting was reduced when learning was closely followed by sleep suggests that sleeping actively stabilizes newly acquired memories, which are then more resistant to interference during subsequent waking (Payne et al. 2012a).
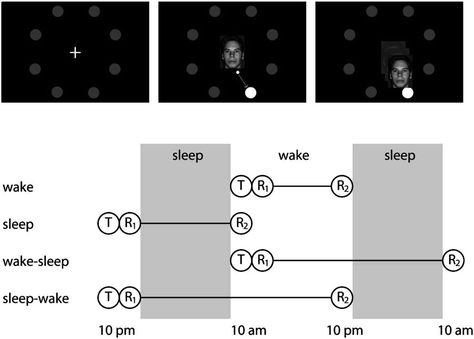
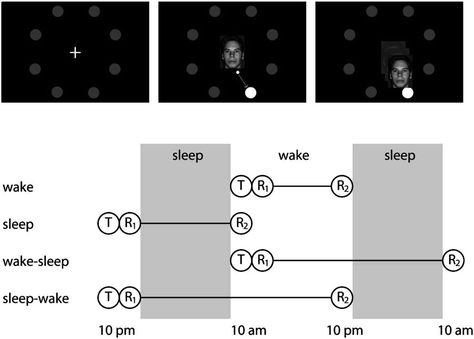
Fig. 4
The design used by Talamini et al. (2008). Participants were first trained to pair faces with spatial locations and were later tested on their memory for these pairings across delays varying in the positioning of sleep
Such extended delays have not only been utilized to rule out interference accounts, but have also provided evidence that sleep may act to restore degraded memories. For example, Backhaus et al. (2007) examined memory for word pairs in children after both a 12-h delay as well as a 24-h delay. Children either initially learned word pairs in the evening and obtained a night of sleep prior to a memory test the following morning (12-h test) as well as the following evening (24-h test) or learned the word pairs in the morning and obtained wakefulness during the day prior to testing that evening (12-h test) as well as the following morning (24-h test). Performance at the 12-h test was better when it followed sleep rather than wake. Further, performance at the 24-h test revealed that when wake followed sleep, memory did not change over the waking interval, remaining at levels seen at initial testing following sleep. This result contributes to the evidence of the active stabilization provided by sleep , which protects the memory from the effects of subsequent interference and decay incurred during wake. Interestingly, performance at the 12-h test, which was worse in those who obtained a day of wakefulness prior to sleep , improved at the 24-h test following the subsequent overnight sleep interval (see Fig. 5). Coupled with studies revealing a similar rejuvenating effect when sleep follows a degrading period of wakefulness within a 24-h time span (Fenn et al. 2003), this finding implicates an active restoration of memory by sleep, allowing one to recover a previously decayed memory in some cases.
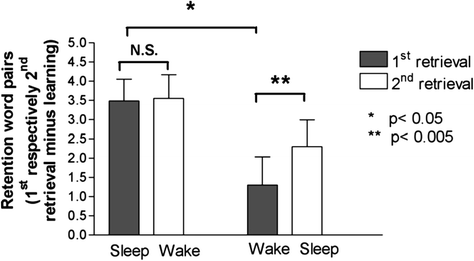
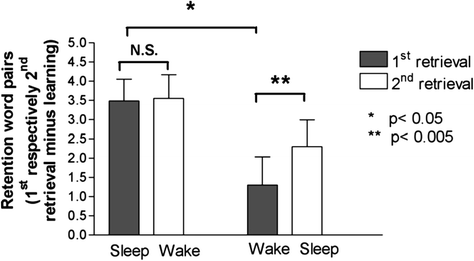
Fig. 5
Children who were trained on word pairs showed no boost in subsequent memory performance when a sleep period came prior to a wake period 24 h later, but when the waking interval proceeded a period of sleep , memory improved at a 24-h test. These results suggest that not only does sleep strengthen memory traces against later interference, but may also play an active role in improving memory (from Backhaus et al. 2007)
5.5 Insight, Gist, and Rule Extraction with Sleep
Because of its role in memory consolidation , sleep is also believed to play an important role in problem solving, supporting the strategic combination of information and experiences from daily life into a useful solution during sleep. Indeed, there are many examples of “sleeping on a problem,” including groundbreaking solutions to some of science’s most perplexing problems arriving through a night of sleep. Without these sleep-induced breakthroughs, we might not know the chemical structure of benzene or have the perfect organization of the periodic table of elements (Stickgold and Walker 2004). Beyond these popular anecdotes, some empirical evidence has begun to surface. Recent studies suggest that the benefit of sleep to memory goes beyond stabilization and enhancement of new knowledge in its veridical form, to restructure and transform that knowledge in a manner that allows for its flexible use (see Payne 2011; Stickgold and Walker 2013 for review). For example, sleep helps modify memories so that inferences can be made (Ellenbogen et al. 2007), insights can be achieved (Wagner et al. 2004), rules and meaning can be extracted (Payne et al. 2009), and complex problems can be solved (Stickgold and Walker 2013).
In one such study, Wagner et al. (2004) found that sleep as opposed to wakefulness was more likely to induce the discovery of a shortcut in a number reduction task. On each of several trials, participants were to reduce a string of eight numbers into a string of seven, given a rule for reducing two of these original numbers at a time. The goal was to find the identity of the last number in the new string. What the participants did not know was that there was a hidden shortcut to obtaining this final number faster; the last three numbers of the new string were actually a mirror image of the previous three, so that once the participant found the second number in the new string, he or she would also know the last number in the string (see Fig. 6). Wagner et al. (2004) had participants undergo an 8-h delay containing nocturnal sleep , nocturnal wakefulness, or daytime wakefulness after training on this task. They found that those who slept after learning were twice as likely as those who stayed awake to find the hidden solution to this task during subsequent testing. Further, even those who did not discover the hidden shortcut benefited from an increased speed of rote responding in the sleep group, a result not found for those in the wake group. Subsequent studies have shown the benefit of sleep to similar rule-dependent memory paradigms as well, such as tasks relying on probabilistic learning of artificial grammars (Fischer et al. 2006) or musical sequences, which appear to depend on the amount of SWS obtained during a period of sleep (Durrant et al. 2011). This evidence suggests that sleep offers an advantage to problem solving after an initial introduction to the problem, an advantage that likely results from the reorganization of memory traces during sleep (Wagner et al. 2004).


Fig. 6
A visual depiction of the number reduction task. Wagner et al. (2004) taught participants to solve puzzles composed of three digits (in this case 1’s, 4’s, and 9’s). Starting from the left side, if the numbers are the same, they enter that number (here, the first two numbers are 1’s, so the participant enters that number). If the numbers are different, they enter the third number possible. They then compare each new number to the next number in the sequence until they reach the final number (in red). Unknown to the subjects, embedded in this task is a hidden shortcut that can be used to speed performance; the last three numbers are always a mirror image of the prior three numbers, with the first number of the mirror (in this case, 9) always being the same as the final answer (Stickgold and Ellenbogen 2008)
Another study examined the role of sleep in enhancing relational memory or the ability to flexibly generalize across existing stores of information. In their study, Ellenbogen et al. (2007) had participants intentionally encode abstract shapes that represented five “premise pairs” (A > B, B > C, C > D, D > E, and E > F). Unknown to the subjects, however, these pairs fit within a larger hierarchy (A > B>C > D>E > F). After a delay of 20 min, 12 h of wake, 12 h of sleep , or 24 h, subjects were tested on not only the premise pairs, which provided the building blocks of the hierarchy (e.g. A > B, B > C, etc.), but also for novel “inference pairs” that had not been previously studied (e.g., B > D, C > E, B > E, etc.; see Fig. 7). The question was whether subjects would be able to correctly infer these relationships without the benefit of direct experience. At retest, all four groups showed nearly identical premise pair retention, but a prominent separation was clear in their abilities to make relational inference judgments. Those in the 20-min group were unable to make the correct inference judgments, with performance nearly at chance. Those in the 12- and 24-h groups displayed a significant improvement in relational memory , but the most striking contrast was the difference in performance between the 12-h period containing sleep compared to the 12-h period of wake. Participants in the sleep group were better at making the most distant, and arguably difficult, inferential judgment (the B > E pair) than the group that remained awake, yet this increase in performance did not correlate with an increase in subjective confidence. This provides evidence that sleep facilitates human inferential ability by enhancing relational memory binding and, further, that this process may occur at an unconscious level (Ellenbogen et al. 2007).
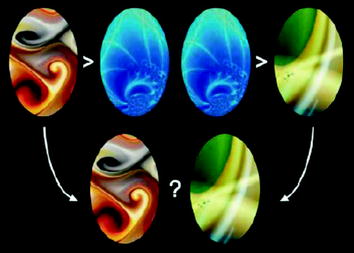
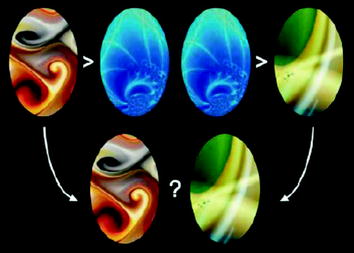
Fig. 7
Example stimuli from Ellenbogen et al. (2007). Through multiple trials, participants learned individual “premise pairs,” for example that the abstract shape with the orange hue is greater than the blue and that the blue is greater than the green. After a delay, they were asked to make inferences about pairings that had never been seen before, yet conformed to the hierarchy (orange > blue > green). The question is whether subjects would be able to correctly infer that the orange shape was greater than the green. Sleeping prior to testing triggered a significant boost in inferential ability (Stickgold and Ellenbogen 2008)
Sleep has also been implicated in the ability to extract meaning or “gist” from previously learned information. A study by Payne et al. (2009) utilized the Deese-Roediger-McDermott (DRM) paradigm, which requires participants to aurally encode lists of words for a later memory test. Each of these lists is composed of words that are semantically related to an unpresented theme or gist word that is commonly falsely recalled as having been included in the original list (e.g., “nurse,” “needle,” and “hospital” are a subset of the studied words that appear on the list for the unpresented gist word “doctor”; Roediger and McDermott 1995). At recall, subjects were asked to record as many words as they could remember hearing. While memory for studied words decreased across delays that included both wake and sleep , the gist words were more often remembered after sleep, showing a numerical improvement. Payne et al. (2009) verified this preferential role for sleep in gist memory in a follow-up nap study. Interestingly, despite previous evidence associating SWS with improved declarative memory performance (Peigneux et al. 2004; Rasch et al. 2007), Payne and colleagues found an inverse relationship between memory for the previously presented words and SWS. Given the semantic relatedness of the words on the studied lists, it was suggested that while SWS may be key for episodic and spatial memory Consolidation (Plihal and Born 1997), it might actually impede semantic memory consolidation . Although it may seem counterintuitive that sleep would promote false memory for gist, this may be a case of sleep rendering memories less accurate but also more useful (a case of seeing the forest through the trees).
6 Memory Consolidation During Daytime Naps
A recent surge in memory research implementing daytime naps not only serves to replicate the results of overnight studies, but also to achieve more insight into the way sleep stages facilitate memory . Naps are used both as a more economic approach to sleep and memory research (because they are typically shorter and therefore cost less), as well as to address inherent confounds that arise in overnight sleep designs. Naps help control time-of-day confounds, with equivalent times of training and testing between groups who sleep and remain awake without the confounds associated with sleep deprivation (see Sect. 7). Additionally, naps minimize concerns about circadian confounds inherent in designs that compare performance in overnight sleep groups to daytime wake groups, which can only be controlled to a certain degree by testing performance across short delays in the morning and evening in typical circadian control groups (e.g., Payne et al. 2008, 2009). While the overnight designs have their own merit as discussed earlier (Sect. 5), nap designs are increasingly employed to practically address the issues stated above and are of sufficient length to differentiate performance between sleep and wake groups, replicating findings from overnight studies.
Due to the typical length of naps in most studies (60–90 min), the potential contributions of particular stages to memory can also be more carefully isolated than in the “early-/late”-sleep paradigm described above (Plihal and Born 1999a, b). This is because a 60- to 90-min nap generally includes Stage 1, Stage 2, and SWS, but little, if any REM sleep. Thus, it is possible to assess only the contribution of NREM sleep to memory consolidation . Using this method, Tucker et al. (2006) found improved paired-associate memory after a nap only containing NREM sleep, but no benefit for a procedural mirror-tracing task. Takashima et al. (2006) additionally found a significant positive correlation between the amount of SWS obtained in a 90-min nap and recognition of pictures viewed before sleep . They also found a negative correlation between memory and hippocampal activation, a finding that grew stronger over a period of 30 days and implicated superior long-term consolidation from the hippocampus to the neocortex after obtaining SWS. Using a virtual maze-learning task, Wamsley et al. (2010) found a positive correlation between dream imagery reported from NREM sleep during a nap and post-nap performance, reflective of processing occurring during the nap. Further, Schmidt et al. (2006) saw increases in spindle density and spectral power in the spindle frequency range after learning difficult paired-associates, compared to easily learned pairs, positively correlating with better post-nap performance. Similarly, Tucker and Fishbein (2008) only saw improvement after a NREM daytime nap when the learned material was strongly encoded during task acquisition, suggesting that the sleeping brain preferentially processes information that perhaps required more neural resources to learn, leading to stronger synaptic connections and potentially higher probability of reactivation during NREM sleep .
6.1 How Much Sleep is Necessary for Memory Consolidation ?
The type of sleep obtained during different nap lengths can also be investigated during daytime sleep without incurring sleep deprivation effects as would be found when manipulating overnight sleep duration. A 2008 study by Lahl et al. found that a brief period of sleep of as little as 6 min facilitated superior recall of a learned list of words compared to remaining awake, but this effect was not as effective as obtaining a nap of 35 min. As a follow-up to this study, Alger et al. (2012) similarly had groups that either achieved a brief nap (10-min), a longer nap (60-min), or remained awake, comparing performance using a bimodal paired-associates task (words paired with sounds). In this study, the findings of Lahl et al. were replicated in that a test of memory for paired-associates immediately after the nap/wake retention period revealed a benefit to performance in the two nap groups, with the longer nap facilitating the memory more than the shorter nap, compared to remaining awake. However, the brief nap’s benefit was found to be temporary and not indicative of true memory stabilization after a stimuli-related interference task was introduced directly following the initial memory test. Memory for the paired-associates degraded in the 10-min nap group, subject to forgetting after interference, while the longer nap group’s memory was relatively protected and preserved. These benefits were seen to persist over a week retention period in the longer nap group, compared to the 10-min nap and wake groups, whose memory decayed similarly. Taking these findings together, it becomes apparent that a nap must include SWS, as opposed to only containing Stages 1 and 2 in the brief 6- and 10-min naps, in order to protect and begin to consolidate the declarative memory trace. In fact, similar results from Schabus et al. (2005) using paired-associates and cued recall performance found that only nappers who obtained SWS during a 60-min nap opportunity showed improvement in the declarative task, compared to those who only had Stages 1 and 2 sleep .
6.2 Naps Facilitate Selective Consolidation
Naps have not only been shown to facilitate veridical memory consolidation , but also to promote preferential memory consolidation , gist extraction, and insight. Saletin et al. (2011) utilized a directed forgetting/remembering paradigm in which an explicit direction of “R” to remember or “F” to forget was given after every presented item. Baseline recognition testing found similar memory performance for cued-to-remember items and cued-to-forget items between groups. However, a nap opportunity of 100 min led to significant selective enhancement of the “remember” items, compared to the wake group, but not for the “forget” items. Similarly, emotional information, compared to neutral, is selectively consolidated during naps. For example, naps have been shown to boost memory for emotional scenes in their entirety (Nishida et al. 2009), as well as memory for specific components of emotional scenes (objects versus associated backgrounds; Payne et al., submitted, see Sect. 8).
Naps can also promote gist extraction, with subjects recalling words that were not actually present in a studied word list, but that represent the general theme or gist of the list. Payne et al. (2009) were able to replicate the extraction of gist found in an overnight design using the DRM task, described earlier, with a 90-min daytime nap. As in the overnight study, they found a significant negative correlation with SWS, concluding that the ability to extract the gist of a list requires the recognition that all the words are related, drawing upon access to semantic memory stores that may not depend on SWS and may in fact be inhibited by it (Payne et al. 2009).
Work by Lau et al. (2010, 2011) has explored relational memory using daytime naps. In a 2010 paper, Lau et al. used an associative inference task in which faces were paired with objects such that one face (A) would be paired with an object (B) in one trial, while the same object (B) was paired with another face (C) in a second trial. After a retention period containing either a nap or wake, those who slept not only preserved the direct AB and BC pairs of faces and objects significantly better than those who remained awake, but also formed superior indirect relational memory of the two faces associated with the same target object (AC pair), similar to relational memory enhancements seen after a full night of sleep (Ellenbogen et al. 2007). In 2011, Lau et al. presented subjects with Chinese characters, which could be grouped by characters containing the same radical or component of the character with a shared semantic component among the group (characters for “mother” or “sister” with the same radical meaning “female”). After a nap, versus no nap, subjects were better able to correctly guess the meaning of a newly presented character with a shared radical of one of the originally learned groups of characters, as well as explicitly state the meaning of a radical. Interestingly, the superior ability to extract this implicit rule was better after sleep regardless of whether the nap occurred immediately after the learning session or a couple hours later.
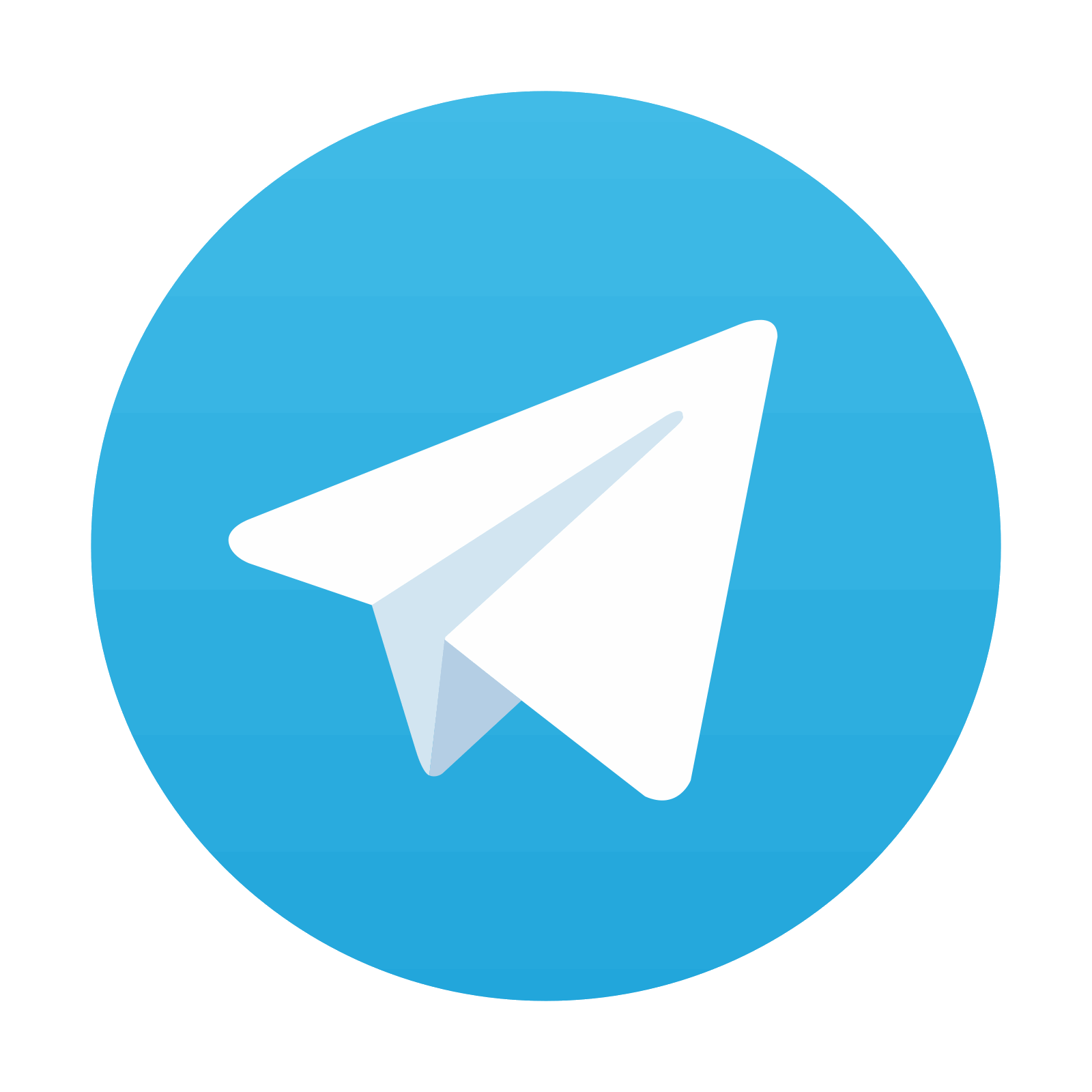
Stay updated, free articles. Join our Telegram channel
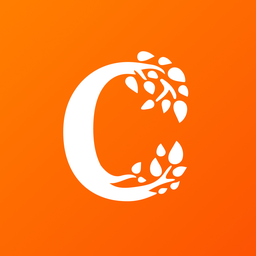
Full access? Get Clinical Tree
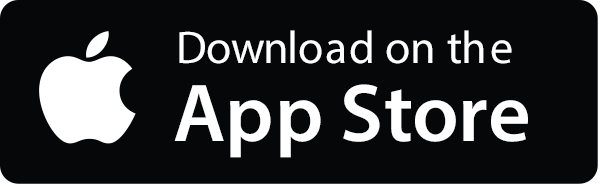
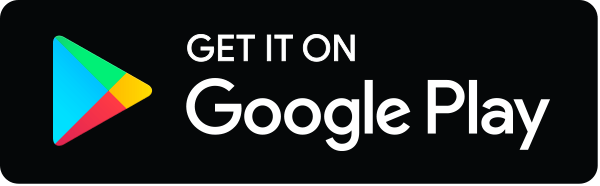