List of abbreviations
CSF
cerebrospinal fluid
SCI
spinal cord injury
MRI
magnetic resonance imaging
CNS
central nervous system
MS
multiple sclerosis
Introduction
Spinal cord injury (SCI) results in severe social–economic problems, because it is observed mostly among younger adults. The incidence of SCI has been reported at 10.5 cases per 100,000 people ( ). This type of injury occurs in young to middle-aged populations due to road traffic accidents, violence, and contact sports. Unfortunately, only 1% of SCI patients experience injuries that fully resolve. Almost half of cases suffer of severe neurological loss (para/tetraparesis, para/tetraplegia) with or without respiratory disturbances ( ).
Several prognostic factors have been proposed according to the previously published studies. But there is still no consensus on which clinical diagnostic tests could be applied to reliably indicate the sub-clinical neurophysiological changes occurring in the spinal cord during the acute phase, and this affects the prediction of cell death and tissue destruction in SCI. Neurological examination provide a general indication of spinal cord neurological function, but are unreliable, especially during the acute phase (first 24 h) after SCI as a result of spinal shock in many patients ( ). MRI provides an accurate anatomical diagnosis, but it is impossible to distinguish neuronal tissue necrosis from post-traumatic edema. Most of the medical decisions are targeting the stabilization of the patients and preventing further injury, but no definitive treatment for the present state of the CNS trauma exists ( ). In these cases, where the real-time injury is the question in clinical diagnosis and prognosis, serum and CSF biomarker evaluation provides another option. A biomarker must be accurate, sensitive, specific, and provide high predictive value. The most widely used biomarker in such cases is S100b, a protein found in cells of neural crest derivation, which can be measured in the serum after disruption of the blood brain barrier ( ). S100b is implicated in a very broad number of CNS conditions, and as such, it is perhaps one of the strongest candidate markers ( ). In case of a CNS injury, accompanied by a nervous tissue and cellular damage, this structural protein is released from nervous cells and its concentration increase extra-cellularly—including CSF and blood. In these cases, S100b could be a potential biomarker of nervous tissue damage ( ).
S100b characteristics
The S-100 protein family constitutes a sub-group of Ca + 2 -binding proteins. The term “S-100” was used because this protein was soluble in 100% ammonium sulfate and refers to a mixture of dimeric proteins consisting of two sub-units termed α and β ( ). Three isoforms are known. S100a (αβ) is found in glial cells and melanocytes, S-100b (ββ) is present in high concentrations in glial cells and Schwann cells of the central and peripheral nervous systems as well as in Langerhans cells and cells of the anterior pituitary gland and S-100a0 (αα), which represents 5% of the S-100 protein in the brain (hippocampus, olfactory cells). The last is found outside the nervous system in slow-twitch muscle, heart, and kidney ( ). The genes encoding the majority of human S100 proteins are organized in a cluster within the chromosomal region 1q21, while some genes coding individual S100 proteins are located in other chromosomal regions, including 21q22 where, in particular, the gene for the S100b protein is located ( ).
S100b is primarily an astrocytic protein and it was first detected in CSF at the acute phase of exacerbation of MS ( ). The majority of astrocytic S100b is located within the cytoplasm, 5%–7% is membrane bound. At least 80%–90% of the total S100b pool is found within the brain, the remainder being located in other non-neuronal tissues. S100b is thought to be involved in a number of Ca + 2 -dependent cellular functions, including protein phosphorylation, enzyme activation, cell proliferation and differentiation, cytoskeletal dynamics, intra-cellular Ca + 2 homeostasis and protection against oxidative injury ( ). In a low concentration it seems to have a neurotrophic effect, it stimulates the growth of neurons, and it increases their survival during development and also during an injury. Higher concentrations might be toxic and evoke cell death. S100b is metabolized and excreted by the kidneys and has a half-life of about 30 min. S100b is found at low levels in healthy patients and remains chemically stable for several hours after sampling ( ).
S100b and CNS pathology
S100b was regarded for almost two decades as specific to the nervous system ( ). Generally, S100b acts like a damage-associated molecular pattern (DAMP), which is released from damaged or activated cells under conditions of cell stress. Furthermore, a more complex role of S100b in the inflammatory processes has been described ( ).
The concept of brain-specific proteins was first proposed in 1965 when S100 was characterized by Moore as a neuronal protein ( ). Many studies followed this conclusion and found a clear role of S100b protein in maintenance and development of CNS. This role of S100b protein could be summarized in the following points ( ):
- •
Regulation of enzymatic activities
- •
Interactions with cytoskeleton proteins
- •
Stimulation of neuronal differentiation, neurite extension activity, and pro-survival effects on neurons
- •
Protection of p53 from thermal denaturation and aggregation; stimulation of p53-dependent cell growth arrest and apoptosis (tumor-suppressor activity); inhibition of p53-dependent transcription activation (tumor-promoting activity) via disruption of the p53 tetramer
- •
Stimulation of astrocyte proliferation and apoptosis
- •
Stimulation of IL-6 secretion by neurons, NO secretion by astrocytes and microglia
- •
Modulation of synaptic plasticity
Over the years, different studies observed a varied grade of correlation between S100b overexpression in neuropathologic states in vivo and trophic/ toxic effects in vitro. For example, overexpression of S100b protein has also been shown in cerebral palsy, Gilles de la Tourette syndrome, Down syndrome, Alzheimer’s disease, schizophrenia, cerebral vasospasm after subarachnoid hemorrhage, astrocytomas, after head injury, and Creutzfeldt–Jacob disease ( ). S100b protein could also represent a useful tool for early detection of intra-ventricular hemorrhage in the post-asphyxia period when clinical examination and cranial ultrasound might still be silent. It is reasonable to hypothesize that S100b accumulates in the extra-cellular space after astrocyte death or due to increased release by activated astrocytes, or after cellular disintegration of the damaged parenchyma. The finding that increased S100b is not accompanied by alterations in other structural markers of neural cell types, such as glial fibrillary acidic protein (GFAP), myelin basic protein (MBP) and neuron-specific enolase (NSE) has been regarded as an indication that S100b is actively released by astrocytes rather than leaked from damaged cells ( ). Under these conditions, the increased accumulation of S100b may become toxic due to its stimulatory effects on nitric oxide production by astrocytes and microglia ( ).
S100b is normally present in cells derived from the neural crest but also from chondrocytes, adipocytes, myoepithelial cells, macrophages, Langerhans cells, dendritic cells, and keratinocytes ( ). Additionally, many studies have suggested extra-cranial sources of S100b, such as following cardiopulmonary bypass surgery, cardiac arrest, carbon monoxide and benzodiazepine poisoning, small and large bone fractures, abdominal injuries, and in fat tissue ( ). Although in trauma patients larger extra-cranial soft tissue and bone injuries led also to an increase of S100b, confounding the interpretation of results, studies focused only on TBI cases revealed a high negative predictive power of S100b. They have found S100b elevation in CSF and peripheral blood after TBI, which is positively correlated with the severity of injury but negatively correlated with outcome. It was concluded that monitoring of S100b levels could contribute to early detection of patients at risk of secondary rises in intra-cranial pressure and subsequent fatal outcome ( ).
Biomarkers in SCI
The acute phase of SCI is evaluated by neurological examination and MRI study. These patients are classified based on image characteristics, severity and need for surgical intervention. In comparison with MRI, neurological findings are most predictive of outcome. As the degree and localization of injured and spared white matter primarily determine functioning after SCI, MRI has limited success as a prognostic tool ( ). On the other hand, injury severity alone does not adequately predict the degree of spontaneous neurological recovery.
Spinal cord trauma causes an acute disruption of the spinal cord parenchyma, which is followed by a secondary axonal degeneration and further degeneration or death of nerve cells by either apoptosis or necrosis, processes that may last from days to weeks. As the spinal cord is surrounded by vessels and CSF, damage to the spinal cord releases proteins and metabolites from the nervous tissue into CSF and blood. The implementation of biomarkers in SCI provides a useful tool supporting clinical decision making in acute SCI, especially in severe injured unresponsive patients ( ; ).
An ideal prognostic CNS biomarker should have all of the following properties: CNS specificity, rapid and profound release into blood or/and CSF after injury, easily assessed, predictability of serious injury from an early sample, correlation of biomarker value with injury severity, of low cost minimally influenced by other factors and reproducible ( ). The most studied biomarkers for SCI patients and their characteristics are summarized in Table 1 .
Biomarkers | Characteristics |
---|---|
Neurofilament proteins
|
|
Glia fibrillary protein (GFAP) |
|
Cleaved Tau protein (C-Tau) |
|
S100b protein |
|
Neuron-specific enolase (NSE) |
|
MAP-2 (microtubule-associated protein 2) |
|
Myelin basic protein |
|
Matrix metalloproteinases (MMPs) |
|
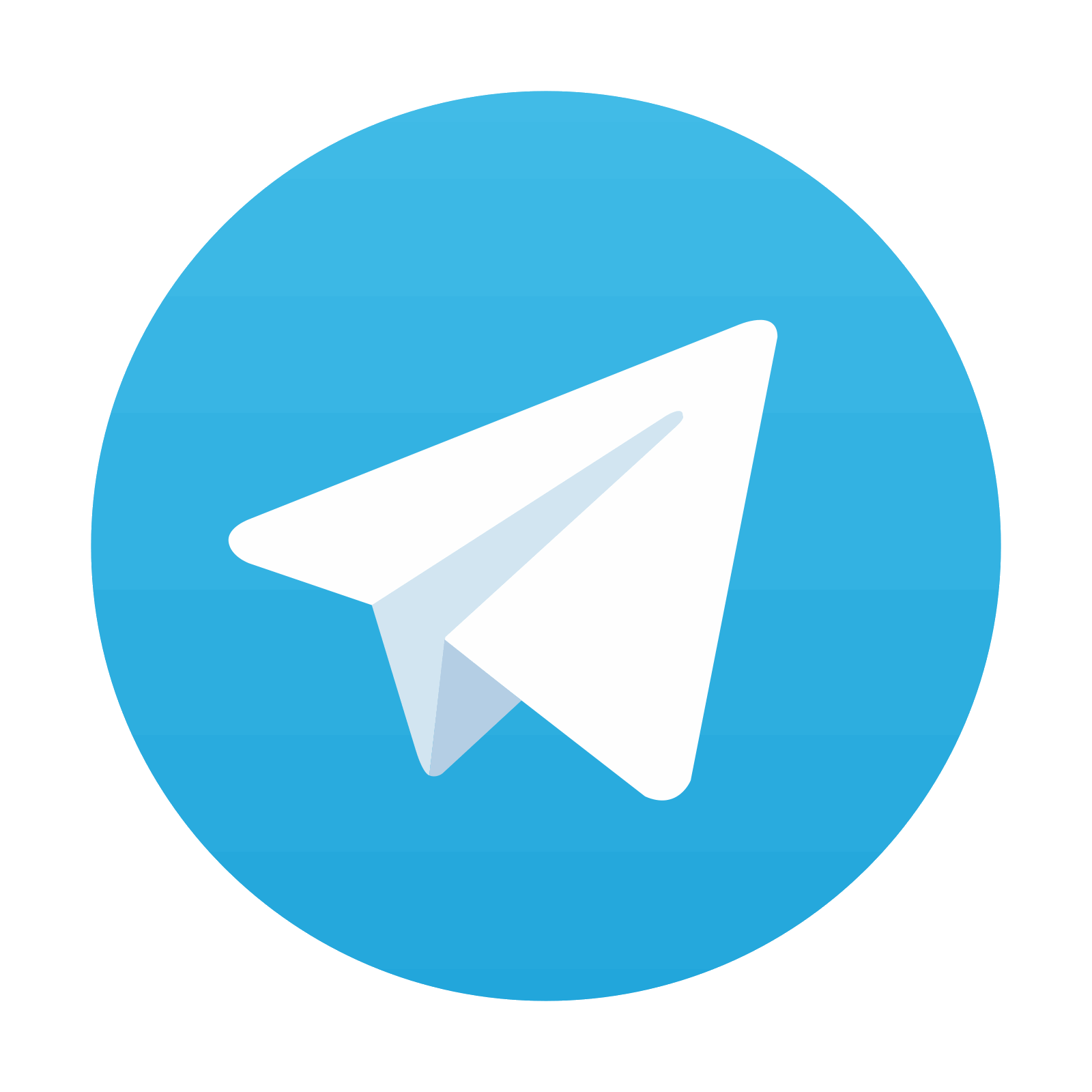
Stay updated, free articles. Join our Telegram channel
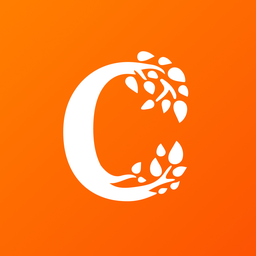
Full access? Get Clinical Tree
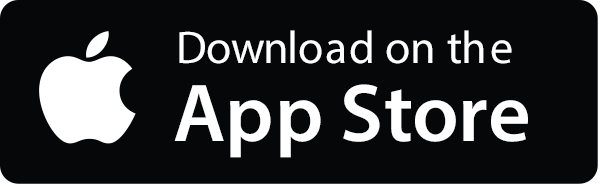
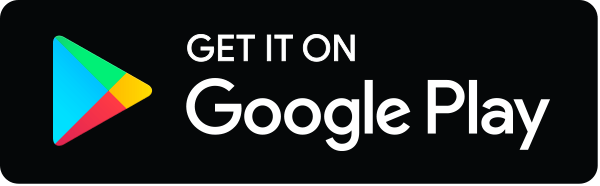
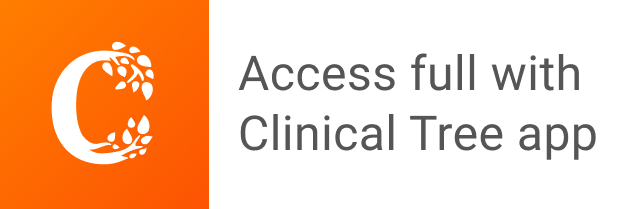