Fig. 13.1
Memory systems and memory stages. a Memory systems. Human memory is most commonly divided into declarative forms, with further subdivisions into episodic and semantic, and nondeclarative forms, subdivided into an array of different types including procedural skill memory. b Developing stages of memory. Following initial encoding of a memory, several ensuing stages are proposed, beginning with consolidation, as well as integration of the memory representation, and translocation of the representation or erasure of the memory. Also, following later recall, the memory representation is believed to become unstable once again, requiring periods of reconsolidation
Declarative memory is the consciously accessible memories of fact-based information (i.e., knowing “what”). Several subcategories of the declarative system exist, including episodic memory (memory for events of one’s past) and semantic memory (memory for general knowledge, not tied to a specific event) [5]. Current neural models of declarative memory formation emphasize the critical importance of structures in the medial temporal lobe, predominantly the hippocampus [6, 7], a structure that is thought to form a contextual retrieval code for neocortically stored information.
In contrast, nondeclarative memory can be regarded as unconsciously acquired learning. The nondeclarative category includes procedural memory (i.e., knowing “how”), such as the learning of habits and motor skills (e.g., playing a piano, athletic sports, surgical skills, etc.), as well as classical conditioning and non-associative learning. The neural basis for nondeclarative learning appears to be more diverse, varying with task characteristics (e.g., motor versus non-motor) [7].
While these categories offer convenient and distinct separations, they rarely operate in isolation in real life. For example, language learning requires a combination of memory sources, ranging from nondeclarative memory for procedural motor programs to articulate speech, to memory of grammatical rules and structure, to aspects of declarative memory for the source of word selection. Such interactions must be kept in mind as we consider the role of sleep in learning and memory.
Memory Stages
Just as memory cannot be considered monolithic, similarly, there does not appear to be one sole event that creates or sustains it. Instead, memory appears to develop in several unique stages over time (Fig. 13.1b). For example, memories can be initially encoded by engaging with an object or performing an action, leading to the formation of a representation of the object or action within the brain. In a subsequent recall stage, memories can be actively recalled or recognized by comparison with the representation. However, the memory continues to evolve between encoding and recall through the process of consolidation. Classically, the term memory consolidation refers to a process whereby a memory becomes increasingly resistant to interference from competing or disrupting factors in the absence of further practice, through the simple passage of time [8]. That is to say, the memory becomes more stable even with time away from the task.
Important, memory stabilization can occur over periods of both wake [9, 10] and sleep [11, 12]. Sleep-dependent consolidation refers to the fact that consolidation is greatest over sleep, compared to wake, for most tasks [13–15]. From this perspective, the enhancement phase of consolidation causes either the active retention of a memory instead of its decay, or the enhancement of a memory over and above its simple maintenance. There is also accumulating evidence that memory consolidation is accompanied by a process of memory integration and generalization [e.g., 16, 17]. Thus, consolidation can be expanded to include more than one phase of post-acquisition memory processing, with each phase occurring in specific brain states such as wake or sleep, or even specific stages of sleep [18].
Although this chapter focuses primarily on the effects of sleep on post-acquisition memory stabilization and generalization, it is important to note that there are additional post-acquisition stages of memory processing that perhaps should also fall under the rubric of consolidation. These include the anatomic reorganization of memory representations (memory translocation), reconsolidation of memory representations following conscious recall (memory reconsolidation), and even the active erasure of memory representations, all of which appear to occur outside of awareness and without additional training or exposure to the original stimuli. It is interesting to note that, while not reviewed here, sleep has been implicated in all of these steps [19–22].
Summary
There are a number of stages of memory processing, which use distinct brain mechanisms to perform separate functions. When multiple classes of memories and the several stages of sleep are combined, one is faced with a truly staggering number of possible ways that sleep might affect memory consolidation.
Behavioral Studies of Sleep and Memory
Evidence of sleep-dependent memory processing has been found in numerous species using a variety of behavioral paradigms. Here, we provide an overview of these studies. The reader is referred to recent reviews for more detail than is provided here [23–25].
Human Studies of Declarative Memory
Much of the early work investigating sleep and memory in humans focused on declarative learning tasks. These studies offered mixed conclusions, some arguing for sleep-dependent memory processing and others against it. For example, De Koninck et al. [26] demonstrated significant increases in post-training REM sleep after intensive foreign language learning, with the degree of successful learning correlating with the percentage increase of REM sleep. Such findings suggest that REM sleep plays an active role in memory consolidation, and that post-training increases reflect a homeostatic response to the increased demands for REM-dependent consolidation.
To the contrary, most recent studies suggest a critical function of slow wave sleep (SWS) in declarative memory consolidation. Perhaps most convincing are studies demonstrating the reactivation of declarative memories during SWS. Rasch et al. [27] taught participants a visuospatial task requiring learning the spatial location of a matrix of images. Learning occurred in the presence of an odor (scent of roses). The experimental odor was re-presented during subsequent SWS for a subset of the participants (Fig. 13.2a). Recall was superior following sleep when the odor present during learning was re-presented during sleep. In a similar study, Rudoy et al. [28] had participants learn a similar visuospatial task, but in this case, each image was paired with a specific sound. Half of these sounds were re-presented during subsequent SWS. Delayed recall was greater for those items for which the associated sound was re-presented during sleep relative to those items for which the associated sound was not re-presented. It is thought that cues presented during sleep, selectively trigger associated memories to be reactivated. Notably, when Rasch et al. [27] presented odors during wake and REM sleep, there was no benefit for subsequent recall beyond that seen in a no-odor (vehicle) control condition (Fig. 13.2b), supporting the specific role of SWS in declarative memory consolidation.


Fig. 13.2
Declarative memory reactivation during slow wave sleep. a Subjects performed a visuospatial learning task in the presence of an experimental odor (scent of roses). During subsequent slow wave sleep, this odor or a non-odor vehicle control was presented. Memory for the visuospatial task was tested following sleep. b Participants recalled more locations following sleep when the experimental odor was re-presented during slow wave sleep compared to vehicle (first panel). Such an effect was not observed if the odor present during slow wave sleep was not also present during learning (second panel), if the odor cue was re-presented during REM (third panel), or if the odor was re-presented during waking (Permissions needed from Rasch et al. [27])
Marshall et al. [29] showed that experimentally boosting human slow oscillations, widespread oscillations between “up” and “down” states that are characteristic of SWS, results in improved memory performance the following day. Following learning of a word-pair list, a technique called transcranial direct current stimulation (tDCS) was used to induce these slow oscillation-like (in this case, 0.75 Hz) field potentials during early delta-rich sleep. The tDCS not only increased the amount of delta sleep during the stimulation period (and for some time after), but also enhanced the retention of these hippocampal-dependent factual memories, suggesting a causal benefit of delta sleep neurophysiology.
Memory enhancement reported in this study is striking in the face of earlier studies that showed memory protection but not enhancement. Notably, this study used a classic paired associates task (semantically related word-pair learning). This task tends to show sleep-dependent memory enhancements [30, 31], while those using semantically unrelated word pairs report sleep-dependent memory protection [32, 33]. The nature of the learning task thus shifts from forming and retaining completely novel associations (dog-leaf) to the strengthening or tagging of well-formed associations (dog-bone) for recall at testing [34].
Sleep’s role in declarative memory consolidation, rather than being absolute, depends on several aspects of the task including task difficulty, future relevance, and emotional salience. More difficult tasks are thought to benefit most from sleep [12, 35]. This may reflect enhanced consolidation for weak compared to strong memories. For instance, learning a second list of word pairs weakens the memory for the first list. Yet, recall of the first list following sleep is greater than when recalled following an equivalent interval of wake while recall of list two differed little following sleep and wake [36].
The benefit of sleep on declarative memories may also be greatest for memories with clear future relevance. Wilhelm et al. [37] demonstrated this preferential effect of sleep by comparing recall after sleep and wake for two groups of participants: one group was informed that they would be asked to recall the learned word pairs in a subsequent session and a second group was not informed of the later recall. Sleep-dependent enhancement in performance was evident for those who expected to recall the words following the delay interval, whereas there was no difference for sleep and wake groups who performed the surprise delayed recall test. Future relevance implied by associating items with a reward (monetary or points) also yields greater performance benefits following sleep compared to unrewarded items [38, 39].
Emotional salience may cue future relevance as well, as memory for items with negative valence is important for self-preservation. A number of studies have illustrated the preferential consolidation of negative emotional memories over neutral memories. Hu et al. [40] found greater recognition of emotionally arousing images after sleep compared to wake while no condition differences were found for neutral images. Moreover, when negative items are embedded in neutral scenes, sleep preferentially enhanced recognition of the negative items relative to the neutral items while the scenes themselves, which were neutral in valence, were uninfluenced by the type of interval (sleep vs. wake) [41]. These behavioral effects have been associated with REM sleep [42]. Likewise, oversleep protection of emotional reactivity to negative images is predicted by the time spent in REM sleep [43].
While these examples highlight when sleep functions to stabilize and enhance veridical memories, as discussed earlier, sleep also functions to generalize declarative memories and integrate them into existing representations [44]. Indeed, the end goal of sleep-dependent memory processing may not be simply the enhancement of individual memories in isolation, but instead, the integration of these memories into a common schema and, by doing so, facilitation of the extraction of universal rules, which may be the basis of generalized knowledge. The most basic demonstration of this comes from studies using the Deese–Roediger–McDermott paradigm in which participants are presented with lists of semantically related words (e.g., BOWL, SPOON, MILK) that lack a critical word representing the gist of the list (e.g., CEREAL). When these lists are learned prior to sleep, recall of the list words is greater than following wake. Notably, sleep also yields greater recall of those critical, “gist” words that were never presented. This suggests that declarative memories are generalized and integrated into the existing lexicon over sleep, thereby allowing the extraction of the more general form of the memory after sleep [17, 45, 46].
Sleep-dependent generalization of declarative memories may provide other cognitive benefits of sleep such as enhancing rule learning, decision-making, and creativity. For instance, it has also been demonstrated that, following initial practice on a numeric-sequence problem-solving task, a night of sleep can trigger insight into a hidden rule and thus improve performance strategy the following morning [47]. Likewise, performance on the Iowa gambling task, a measure of affectively guided decision-making, becomes more optimal after sleep as subjects gain more insight into the statistical probabilities of the four decks of cards from which they must choose [48]. Integrating new memories with prior representations may also yield novel, creative solutions [49, 50].
Taken as a whole, these studies suggest a rich and multifaceted role for sleep in the processing of human declarative memories. While SWS is associated with learning of nonemotional veridical memory processing, REM sleep appears to contribute to changes in the emotional memory representation. How sleep facilitates memory generalization and the function of sleep in translocation and reconsolidation of declarative memories are a matter of ongoing research.
Human Studies of Procedural Memory
The benefit of sleep has been demonstrated across a wide variety of procedural tasks spanning the visual, auditory, and motor systems. In this section, we review evidence of procedural memory improvements following sleep as well as possible limitations.
Motor Skill Learning
Motor skill learning is defined as the process by which movements are more quickly and accurately executed after practice [51, 52]. Motor skills are often broadly classified into two forms: motor sequence learning (e.g., learning a piano scale) and motor adaptation (e.g., learning to use a computer mouse) [53].
The movement sequencing task, a common task for measuring sequence learning, requires that subjects repeatedly tap a sequence of response keys as quickly and accurately as possible. With training, movement time decreases, indicating that learning has occurred. A night of sleep can trigger significant performance improvements in speed and accuracy on a sequential finger-tapping task, while equivalent periods of time awake provide little to no benefit [15, 33]. These sleep-dependent benefits appear to be specific to both the motor sequence learned [54, 55] and the hand used to perform the task [56]. Furthermore, the amount of overnight learning expressed the following morning correlated positively with the amount of stage 2 NREM sleep, particularly late in the night (Fig. 13.3) [15]. This late-night stage 2 NREM window corresponds to a time when sleep spindles, a defining electrophysiologic characteristic of stage 2 sleep, reach peak density [57]. Interestingly, spindles have been shown to increase following training on a motor task [58, 59] and are associated with cellular plasticity as discussed later in this chapter.


Fig. 13.3
Sleep-dependent motor skill learning in the human brain. a Wake 1st: After morning training (10 am, light blue bar), subjects showed no significant change in performance when tested after 12 h of wake time (10 pm, light blue bar). However, when tested again following a night of sleep (10 am, dark blue bar), performance had improved significantly. b Sleep 1st: After evening training (10 pm, light blue bar), subjects displayed significant performance improvements just 12 h after training following a night of sleep (10 am, dark blue bar), yet expressed no further significant change in performance following an additional 12 h of wake time (10 pm, dark blue bar). c Sleep stage correlation: The amount of overnight improvement on the motor skill task correlated with the percentage of stage 2 NREM sleep in the last (fourth) quarter of the night (stage 2 NREM4). Asterisk, significant improvement relative to training; error bars, standard error of the mean (SEM)
A more detailed analysis of the performance improvements on the motor sequencing task after sleep provides insight into how improvements come about [60]. Prior to sleep, individual key-press transitions within the sequence are uneven (Fig. 13.4a, light circles), with some transitions seemingly easy (fast) and others problematic (slow), as if the entire sequence was being parsed into smaller subsequences during initial learning (a phenomenon termed chunking [61]). Surprisingly, after a night of sleep, the problematic slow transitions are preferentially improved, while transitions that were already mastered prior to sleep did not change (Fig. 13.4a, dark circles). In contrast, if subjects were retested after an 8-h waking interval across the day, no such improvement in the profile of key-press transitions, at any location within the sequence, was observed (Fig. 13.4b). These changes suggest that the sleep-dependent consolidation process may involve the unification of smaller motor memory units into one single memory element by selectively improving problem regions of the sequence. This overnight process would therefore offer a greater degree of performance automation and effectively optimize skill speed throughout the entire motor program.


Fig. 13.4
Single-subject examples of changes in transition speeds. Within a five-element motor sequence (e.g., “4-1-3-2-4”), there are four unique key press transitions: (1) from 4 to 1, (2) from 1 to 3, (3) from 3 to 2, and (4) from 2 to 4. a The transition profile at the end of training before sleep (light circles) demonstrated considerable variability, with certain transitions being particularly slow (most difficult; “problem points”), whereas other transitions appear to be relatively rapid (easy). Following a night of sleep (dark circles), there was a specific reduction (improvement) in the time required for the slowest problem point transition. b Similarly, at the end of training before a waking interval, transition profiles were uneven (light circles), with some particularly slow transitions (“problem points”) and other relatively fast transitions (easy). However, in contrast to post-sleep changes, no change in transition profile was observed following 8 h of wakefulness (dark circles)
The role of sleep in motor adaptation is less clear. Doyon and colleagues [62, 63] compared off-line changes in motor sequence learning to off-line changes in visuomotor adaptation. To measure adaptation, subjects used a joystick to move a cursor on the screen to a target. After practice, mapping between the joystick space and the cursor on screen was inverted. Movements that were initially slow and inaccurate under inverted mapping conditions became faster and more accurate over time as participants adapted to the visuospatial shift. While replicating sleep-dependent performance changes on the motor sequence learning task, Doyon and colleagues failed to find preferential changes in performance over sleep on the motor adaptation task. Rather, motor adaptation improved equally over intervals with wake and sleep. These results are in contrast to an earlier study by Huber et al. [64]. Moreover, a rather consistent benefit of sleep has been observed for learning on a mirror tracing task which, like the visuomotor adaptation task of Doyon, requires learning of novel mapping between motor output and visual input. For example, Plihal and Born [65] found improvements in mirror tracing performance following late-night sleep, where REM and stage 2 non-REM dominate, even when early-night sleep was deprived. No such improvements were observed when participants had SWS-rich early-night sleep and late-night sleep was deprived. Moreover, Tamaki [66] found increased sleep spindle amplitude and duration after learning a mirror-tracing task, and this increase was associated with over-sleep improvements in performance.
Conflicting results across studies of motor skill consolidation may reflect limitations on sleep-dependent consolidation of motor skills. For instance, motor skill consolidation over sleep is biased by task difficulty. Kuriyama investigated the effects of increasing task complexity on sleep-dependent motor learning [60]. Subjects trained on a variety of task configurations involving either a short or long motor sequence performed either with one hand (unimanual) or coordinated between two hands (bimanual). Interestingly, the more complex the task became (long sequence, bimanual), the greater the overnight, sleep-dependent memory enhancement. This would indicate that, as task difficulty increases, the overnight sleep-dependent process responds with even greater performance improvements. Consistent with this, sleep was reported to benefit motor sequence learning for adults who were trained to an intermediate level of performance prior to sleep, whereas there was no benefit of sleep for experts [67].
Additionally, performance benefits from sleep are greater when participants are aware of the information to be learned [68]. This was demonstrated in a study comparing implicit motor sequence learning (subjects were not aware that finger responses to visual cues were in a sequence) under conditions which were either contextual—the sequence was embedded in a unique repeating context—or non-contextual. Sleep-dependent consolidation was present for the implicit contextual learning condition but not the non-contextual condition. Given that contextual learning is known to engage the hippocampus [69], we interpret these results as demonstrating the role of the hippocampus in consolidation of motor skills.
Taken together, overnight sleep has been shown to benefit motor skill performance, a benefit primarily associated with stage 2 NREM sleep and associated sleep spindles. Discrepancies across studies may be explained by limitations on this process with preferential consolidation for difficult tasks and those that engage the hippocampus [reviewed in 24].
Perceptual Learning
Karni et al. [70] have demonstrated that learning on a visual texture discrimination task improves significantly following a night of sleep. Furthermore, they established that selective REM, but not NREM sleep appears essential for these performance gains. Using the same task, Stickgold et al. [71] have shown that these enhancements are specifically sleep- and not time-dependent. Specifically, performance gains were correlated positively with the amount of both early-night SWS and late-night REM sleep, and the product of these two sleep parameters explained over 80 % of intersubject variance, an incredibly strong correlation.
Performance on a repeated visual search task also improves with sleep. In this task, participants are presented with arrays of L’s in various orientations and must find a hidden T. When displays are repeated, although unbeknownst to the observer, reaction time decreases. In spite of the implicit nature of learning, individuals with medial-temporal lobe lesions (including the hippocampus) fail to learn this task [72]. Geyer et al. [73] demonstrated sleep-dependent improvements on this task, as measured by reduced reaction times to repeated displays further supporting a role of the hippocampus in sleep-dependent consolidation even in the procedural domain.
The Weather Prediction Task is a probabilistic category-learning task in which participants learn to predict the “weather” from a set of cards, each of which has a learned probability of “sun” or “clouds” [74]. Djonlagic et al. [75] found that a group of subjects who slept after observing responses on the task made significantly more accurate weather predictions than a group who stayed awake. Here too, the performance benefits for the sleep group varied by the level of encoding: Those participants for whom the task was less difficult (high accuracy) before sleep showed less improvement than those for whom the task was more difficult (intermediate accuracy). Moreover, when the study was repeated and subjects were required to learn the probabilities through feedback, now sleep had no effect on performance. Given that observational learning is hippocampus-dependent while feedback-based learning of this task is associated with the striatum [e.g., 76], the authors interpreted these results to suggest that sleep may consolidate hippocampal-based learning but not memories encoded in the striatum.
Summary
In summary, delayed off-line learning of many perceptual and motor skills appears to develop during overnight sleep and not across equivalent time periods awake. Such changes have been associated with stage 2 NREM and sleep spindles that are predominantly found in this sleep stage. However, the function of hippocampal-dependent reactivation in SWS is suggested to play a parallel role. How these functions interact across sleep is a matter of ongoing research [e.g., 24, 77]
Animal Studies
Studies using animal models have provided evidence for the role of sleep in primarily hippocampus-dependent tasks. Training on both spatial and shock avoidance tasks triggers alterations in sleep-stage characteristics [78–82], suggesting, as in humans, a homeostatic response to increased demands on sleep-dependent consolidation mechanisms. In one such study, the magnitude of change in sleep architecture following learning demonstrated a strong relationship to initial performance during acquisition, with animals that learned quickly showing the largest change in sleep structure, while those that learned poorly showed relatively little [83].
The benefit of sleep after learning on subsequent performance has been demonstrated in a range of species including non-human primates [84], cats [85], rats [86, 87], starlings [13, 88], honeybees [89], and fruit flies [90]. In one such study, Inostroza et al. [87] examined memory for object-place pairs in rats following an 80-min interval that either contained sleep or was spent fully awake. Memory for the location of objects was greater following sleep, whereas animals performed at chance if kept awake after encoding the object location (Fig. 13.5a). Moreover, sleep did not benefit the memory for a novel object recognition task, which is thought to be encoded independent of the hippocampus (Fig. 13.5b).


Fig. 13.5
Sleep-dependent learning in rats. a Animals learned an object-location task in the morning followed by normal sleep (Sleep) or sleep deprivation (S–Deprivation). The Wake condition took place in the evening when wakefulness is typical (i.e., not sleep deprived). Animals were significantly more likely to identify the misplaced object following sleep compared to both the sleep-deprivation and wake conditions as measured by the discrimination ratio (time spent at novel minus time spent at familiar divided by total exploration time). b In the novel-object recognition task, thought to be encoded independent of the hippocampus, animals were probed for their memory of a new object in the arena following sleep. There was no difference in discriminability for the novel object following sleep compared to the sleep-deprivation and wake conditions (Permissions needed from Inostroza et al. [87])
Memories are not only protected over sleep but enhanced as demonstrated in starlings, a songbird known for its ability to imitate the sounds of other birds. If two songs are learned in parallel, the memories interfere with one another as seen by impaired performance following an interval spent awake. However, when birds slept following the exposure to the interfering songs, performance improvements were observed, even when sleep followed a significant waking delay. These results suggest that sleep actively restored and enhanced the song memories. Additionally, when interference was presented following sleep, it did not impair performance for the song learned prior to sleep. Collectively, this work in song birds, similar to observations in humans [91], suggests that learning is actively consolidated and enhanced over sleep, leaving it more resistant to interference after sleep.
Summary
Taken as a whole, behavioral studies in humans and animal models leave little doubt that sleep plays a critical role in post-training memory consolidation. While both declarative and procedural memories have been shown to improve following sleep compared to wake, these memory systems appear to require subtly different sleep stages or even sleep-stage time windows, for consolidation and overnight improvement. With such evidence, it is important to consider the impact of little to no sleep as measured by studies of napping and sleep deprivation.
Sleep Deprivation
Reinforcing the role of sleep in memory consolidation is a wealth of literature illustrating the converse that sleep deprivation results in impaired declarative and procedural learning [80, 89, 92–100]. For example, total sleep deprivation (38 h awake) [101] and sleep restriction (overnight sleep reduced to 4 h) [102], both yield performance impairments on a number of cognitive tasks including declarative learning.
However, many of these studies have been legitimately criticized for a failure to control for general effects of sleep deprivation on performance [103, 104]. Retesting in a sleep-deprived state may mask evidence of successful consolidation due to lowered alertness and attention. Alternatively, the increased stress of prolonged wakefulness, rather than the lack of sleep itself, may be the cause of unsuccessful consolidation.
More recent studies in both humans and animals have, however, demonstrated that impaired performance can still be seen several days after the end of sleep deprivation, when alertness and attention have returned to normal [105]. In addition, selective deprivation of specific sleep stages inhibits memory consolidation [98, 106], making arguments of sleep deprivation-induced stress relatively untenable, since the stress effects would have to be uniquely produced by deprivation of specific sleep stages during specific time windows following training.
Napping
Given this clear evidence that sleep is advantageous and sleep deprivation is disadvantageous for learning, several studies have asked the question “how much sleep is enough?” These studies have consistently revealed that a nap is sufficient for sleep-dependent changes in declarative [31, 107] and procedural memories [108–112]. Even a very brief nap of 6 min has been reported to increase recall of a declarative, word-list learning task [113]. Nonetheless, more sleep is better. Mednick and colleagues have shown that a 30- to 60-min daytime nap between repeated administrations of a texture discrimination task protects learning from the performance deterioration that is seen when subjects stay awake. If a longer nap period is introduced, ranging from 60 to 90 min and containing both REM sleep and NREM SWS, performance not only returns back to baseline but may be enhanced.
Sleep-Dependent Brain Plasticity
Memory formation depends on brain “plasticity”—lasting structural and functional changes in a neuron’s response to a stimulus. The behavioral studies described above indirectly suggest a role of hippocampal plasticity in sleep-dependent memory consolidation, but recent direct evidence of sleep-dependent plasticity greatly strengthens this claim. In this section, we consider a wealth of data describing sleep-dependent brain plasticity at a variety of different levels in both animals and humans, complementing evidence of sleep-dependent changes in behavior.
Neuroimaging Studies
Modification of Post-training Sleep and Brain Activation
Several studies have investigated whether initial daytime training is capable of modifying functional brain activation during later sleep episodes. Based on earlier animal findings (described below), neuroimaging experiments have focused on whether the signature pattern of brain activity elicited while practicing a memory task actually re-emerges, or is “replayed” during sleep.
Maquet and colleagues have shown that patterns of brain activity expressed during motor skill training reappear during subsequent REM sleep, while no such change in REM sleep brain activity occurs in subjects who received no daytime training [114] (Fig. 13.6). Furthermore, these researchers have gone on to show that the extent of learning during daytime practice exhibits a positive relationship to the amount of reactivation during REM sleep [115]. Training on a hippocampus-dependent virtual maze task during the day has similarly been associated with a subsequent increase in hippocampal reactivation in NREM SWS [116], with the magnitude of reactivation correlating with performance on the task the following day, suggesting a function for neuronal replay. Such findings suggest that it is not simply experiencing the task that modifies subsequent sleep physiology, but the process of learning itself, and that this reactivation can lead to next-day behavioral improvements.


Fig. 13.6
Task-dependent reactivation of human brain activity during REM sleep. Statistical activation maps of different experimental contrasts. Maps are displayed at six different brain levels (from 16 mm below to 64 mm above the bicommissural plane), superimposed on the average magnetic resonance imaging (MRI) scan of subjects. All maps are thresholded at p < 0.001 (uncorrected), except for A, which is thresholded at voxel-level-corrected p < 0.05. a Brain regions activated during daytime performance of the motor skill task (Task–Rest). b Brain regions activated during subsequent REM sleep in subjects who received daytime training (REM Sleep Training–Rest); note considerable overlap with daytime task-dependent activity patterns. c Brain regions activated during REM sleep in subjects who did not receive any daytime training (REM Sleep No Training–Rest) (Reproduced with permission from Maquet et al. [114])
Overnight Reorganization of Memory Representations
An alternative approach to investigate sleep-dependent plasticity is to compare patterns of brain activation after a night of sleep. In contrast to measuring changes in functional activity during sleep, this approach aims to determine whether there is evidence that the neural representation of a memory has been reorganized following a night of sleep and whether this reorganization differs from that which may occur over wake.
With the behavioral characteristics of sleep-dependent motor sequence learning well established, Walker et al. were the first to examine the neural basis of sleep-dependent changes in learning by investigating differences in brain activation before and after sleep using functional magnetic resonance imaging (fMRI) [117]. Following a night of sleep, relative to an equivalent intervening time period awake, increased activation was identified in motor control structures of the right primary motor cortex (Fig. 13.7a) and left cerebellum (Fig. 13.7b)—changes that likely allow faster motor output to the trained, left hand, and more precise mapping of key-press movements post-sleep. There were also regions of increased activation in the right medial prefrontal lobe and hippocampus (Fig. 13.7c, d), structures that support improved sequencing of motor movements in the correct order. In contrast, decreased activity post-sleep was identified bilaterally in the parietal cortices (Fig. 13.7e), possibly reflecting a reduced need for conscious spatial monitoring, and throughout the limbic system (Fig. 13.7f–h), indicating a decreased emotional task burden. In total, these results suggest that sleep-dependent motor learning is associated with a large-scale plastic reorganization of memory throughout several brain regions, allowing skilled motor movements to be executed more quickly, more accurately, and more automatically following sleep.


Fig. 13.7
Sleep-dependent motor memory reorganization. Subjects were trained on a sleep-dependent motor skill task and retested 12 h later, either following a night of sleep or following intervening wakefulness, during a functional MRI (fMRI) brain scanning session. Scans after sleep and wakefulness were compared (subtracted), resulting in regions showing increased fMRI activity post-sleep (in red/yellow; a–d) or decreased signal activity (in blue; e–h) post-sleep, relative to post-wakefulness. Activation patterns are displayed on three-dimensional rendered brains (top panel of each graphic), together with corresponding coronal sections (bottom panel of each graphic). Following sleep, regions of increased activation were identified in the right primary motor cortex (b), the left cerebellum (a), the right hippocampus (c), and the right medial prefrontal cortex (d). Regions of decreased activity post-sleep were expressed bilaterally in the parietal lobes (e), together with the left insula cortex (f), left temporal pole (g), and left frontopolar area (h), all regions of the extended limbic system. All data are displayed at a corrected threshold of p < 0.05
Debas et al. [62] contrasted changes in neural activation following sleep and wake for a motor skill learning task and a motor adaptation task. Consistent with their behavioral observations described above, there was an increase in activation in the primary motor cortex, the cerebellum, and the striatum for the motor sequence learning task following the off-line interval, with the activation of the striatum specifically modulated by sleep. However, there were no sleep-specific changes in neural activation patterns in conjunction with the motor adaptation task, consistent with the observation of limited over-sleep change in performance for this task.
Visual skill learning is also associated with underlying cortical plasticity. Post-sleep performance was associated with significantly increased activity not only in the primary visual cortex, but also in several downstream visual processing regions following sleep, at the occipital–temporal junction and in the medial temporal and inferior parietal lobes (regions involved in object detection and identification) [118]. These findings strengthen the claim that a night of sleep reorganizes the representation of a visual skill memory, with greater activation throughout the visual system following sleep likely offering improved identification of both the visual stimulus form and its location in space.
While most studies of over-sleep neural reorganization have focused on procedural learning, the neural basis of declarative memory consolidation has more recently been investigated. Takashima et al. [119] examined the long-term effects of a nap following the encoding phase of a picture recognition task. Picture recognition and the associated neural correlates were measured following a delay of 1, 2, 30, and 90 days. Following a delay of 1 day, the amount of time spent in SWS correlated positively with recognition performance and negatively with hippocampal activity during correct recognition. With time, recognition was associated with a decrease in the hippocampus activation coupled with a corresponding increase in ventromedial prefrontal cortex activation. This result is interpreted to suggest that SWS is associated with transfer of memories from the labile storage in the hippocampus to a more permanent representation in the cortex.
Overnight reorganization of emotional declarative memories has been the focus of many recent studies [120–122]. Similar to non-emotional declarative memories, memory for negative images following sleep is associated with less diffuse hippocampal activation. Specifically, Payne and Kensinger [120] examined changes in neural activity associated with an emotional picture recognition task following retention intervals with sleep or wake. Patterns of neural activation associated with successful recognition were distinct following sleep versus wake. After an interval of daytime wake, successful recognition of the negative images was associated with activation in a broad network including the lateral prefrontal, parietal, and medial temporal lobes (including the hippocampus). In contrast, recognition performance following sleep was concentrated to a smaller network including the ventromedial prefrontal cortex, left amygdala, and cingulate gyrus.
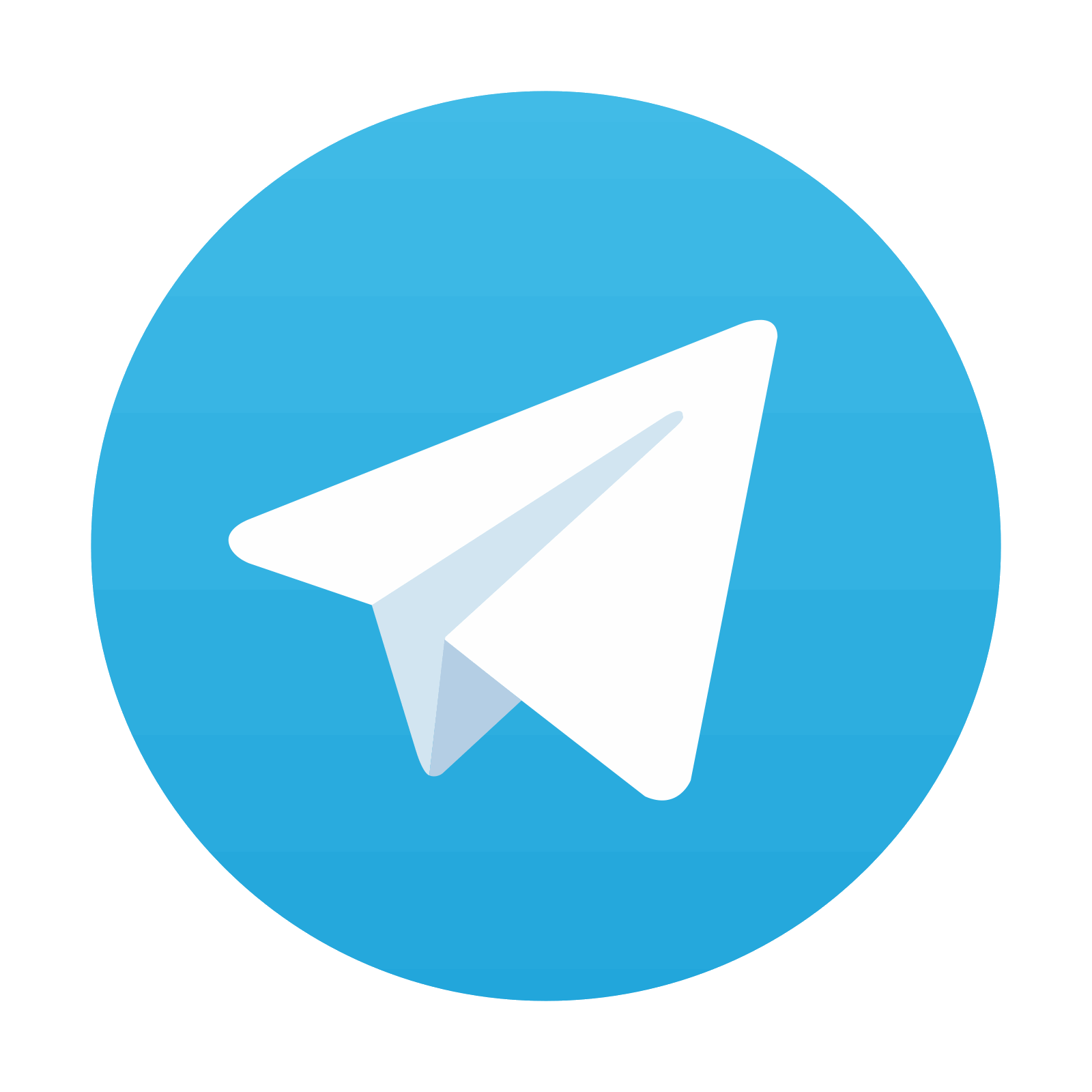
Stay updated, free articles. Join our Telegram channel
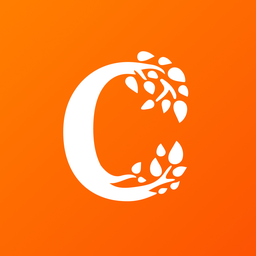
Full access? Get Clinical Tree
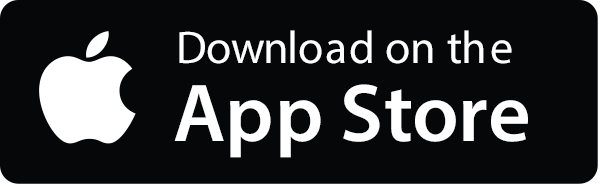
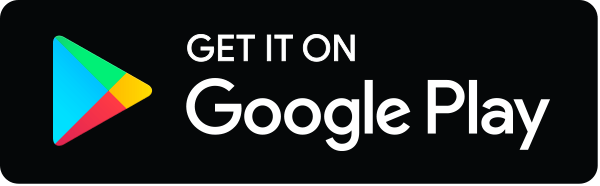