Fig. 57.1
Major frameworks for modeling sleep-wake rhythms [37]. S/W and Temp denote sleep-wake and temperature rhythms, respectively. a Two process framework integrating homeostatic and circadian regulations. b Multioscillator framework consists of interacting multiple oscillators, where line thickness indicates strength of coupling. For more detail explanation, see the text
In this chapter, model constructions based on the frameworks shown above and their characteristics are reviewed comparatively. Then, recently acquired knowledge of neural regulatory mechanisms of sleep-wake rhythm is summarized. The detailed neural network models of sleep-wake regulation are also reviewed. These models constructed within various frameworks could serve for testing reality of a physiologist’s idea and for exploring a possible mechanism at various levels underlying pathological states of sleep-wake rhythm.
Human Sleep Models
Models Based on Interactions Between Homeostatic and Circadian Control of Sleep-Wake Rhythm
Homeostatic control of sleep-wake rhythm can be intuitively understood; because it is usually experienced that sleep deficit is compensated by a deeper and/or longer recovery sleep. This intuition is well embodied in the two process model [3, 10], which consists of periodic C processes and an exponentially rising-decaying S process. C process works as a reflecting boundary against S process: the S process rebounds when it reaches the C process. As many reviews of the two process model have already been written by Borbély and his colleagues (e.g., [23]), here we summarize aspects of the model not typically covered elsewhere. The two process model was developed based on the empirical data obtained under short-term sleep deprivations [3]. Namely, the S process represents a build-up of slow wave electroencephalographic activity (SWA) during wake, and its decay during sleep. The shape of C process is determined by a level of SWA and sleep-onset/wake-onset timing [3, 10]. The two process model provides an intuitively tractable structure that enables researchers to test their ideas by manipulating the parameters and model operations: changing the gap between C processes (short and long sleeper), skipping the reflection of S process at the intersection of two processes (sleep deprivation and shift work), reducing build-up of S process (napping), and so on [23]. For 30 years after the development of the two process model, its simplicity and utility have led to its extensive acceptance as a symbolic conceptualization of the integration of homeostatic and circadian controls.
One possible modeling framework of homeostatic control is a negative feedback control. According to the physiological finding that thermoregulation and sleep control may be integrated in the preoptic/anterior hypothalamic area [24], a thermoregulatory model of sleep control was developed. In this context, the mathematical model consists of two circadian oscillators and two negative feedback loops for thermoregulation, one of which is mediated by sleep-wake rhythm [25, 26]. This model has variables mimicking “sleepiness” in addition to sleep-wake patterns, the hypothalamic temperature, and a set-point of thermoregulation. This model can predict behavior of sleepiness and temperature under various situations such as sleep deprivation and shift work [25, 26]. A possible physiological origin of biphasic sleepiness was explored in light of this model structure [27]. This is another mathematical modeling of the interactions between homeostatic and circadian controls of sleep-wake rhythm.
Prediction of alertness and cognitive performance under various environments is one of major targets of recent modeling [11, 12, 28]. Most of the models generally share the framework of the two process model, although the interactions between the homeostatic and circadian regulations are differently expressed. In addition, another component such as sleep inertia (transitional state of lowered arousal occurring immediately after awakening) can be included as in a three process model [11, 29]. The distinct structure in these models is nonlinear mutual interactions between the homeostatic and circadian regulations, in contrast with a unidirectional effect of C process on S process in the original two process model . The nonlinear interactions were added to reflect results from the forced desynchronization protocol which attempts to separately evaluate the homeostatic and circadian regulations of wide variety of physiological and cognitive variables [30–32]. Borbély and his colleagues have been extending the structure of the two process model by including circadian and ultradian REM-NREM oscillators in a composite form [13], or by implementing a REM-NREM regulatory mechanism [33]. Daytime vigilance can also be simulated by the extended model [14, 34].
The two process model also has a sophisticated structure as a nonlinear oscillator [35]. Due to this structure, it can easily accommodate a wide variety of behavior in sleep-wake rhythm. Actually, the different types of internal desynchronizations can be understood as a bifurcation of the dynamics of the two process model [35, 36]. The beauty of the two process model may be in its simple and abstract structure with no redundancy, which deserves more investigation [37].
Models Based on Interacting Multiple Oscillators
Discovery of dissociation between temperature/melatonin and sleep-wake rhythms (internal desynchronization) in a situation without any time cues such as light and feeding (free-run) prompted development of multioscillator models [1, 2, 15, 18]. As shown in Fig. 57.1b, the fundamental framework of a multioscillator model is two mutually interacting oscillators: one drives temperature and melatonin rhythms (tentatively called Osc I), generally reflecting the actions of the suprachiasmatic nucleus (SCN), and the other controls the sleep-wake rhythm (tentatively called Osc II) that is often represented by plural oscillators [16, 18]. In addition, Osc I is photo-responsive, and exerts a stronger effect on Osc II than the opposite direction. This organization is assumed because of the robustness of Osc I relative to Osc II. These models simulate the behavior of circadian rhythms in a free-run situation including the internal desynchronization and the non-monotonic relationship between the sleep-onset phase and the corresponding sleep length [38].
The physiological reality of the multioscillator framework and the homeostasis-rhythm interaction framework has been disputed [39]. Currently, prediction of alertness and cognitive performance prefers the latter framework. However, it is still argued how homeostatic and circadian regulations interact with each other [11, 34]. It should be noted that the rigid experimental and theoretical separations between homeostatic and circadian regulations are difficult to be done, because a stable limit cycle, which is a general model of circadian oscillator, is characterized by an ability to restore its oscillatory orbit against external perturbations just like homeostasis .
In the multioscillator framework, a physiological origin of Osc II has not yet been identified, in contrast with Osc I (which represents the SCN). This fact may weaken its physiological reality. Honma and his colleagues have tried to map Osc II onto a physiologic process or structure, and to clarify its dynamics [40]. In their experiment, the rest-activity cycle (schedule) of a subject was advanced by 8 h relative to the spontaneous sleep-wake rhythm under the free-run condition, and the reentrainment of the melatonin rhythm was traced after release from the schedule. It took several days for the sleep-wake rhythm to be reentrained to the melatonin rhythm after an 8-day advanced schedule. In contrast, after a 4-day schedule, the sleep-wake rhythm immediately caught up with the melatonin rhythm in most subjects. These results suggest that the sleep-wake rhythm is underlain by an oscillator (presumably Osc II), which could be entrained by the rest-activity schedule (i.e., a nonphotic entrainment) . The nonphotic entrainment of Osc II was found to take 4 days or longer; implying that there is a critical period for the entrainment of Osc II. In other words, an adaptive feedback mechanism from the rest-activity cycle to the circadian oscillators is suggested to exist. There is physiological evidence supporting such adaptive feedback mechanisms [41–44].
Existence of the nonphotic as well as photic entrainment mechanisms leads to the multioscillator model with feedbacks [17, 18, 45]. This model can simulate behavior of sleep-wake rhythm under transmeridian flight and shift work where the photic and nonphotic entrainment mechanisms should interact with each other. Incorporating the thermoregulatory model of sleep control the multioscillator model could raise its applicability under diverse situations [46].
Neural Network Models of Sleep-Wake Rhythm
Most of the aforementioned models have abstract structures in which the details of mechanisms at neural and molecular levels are not explicitly represented. Their applicability in understanding the mechanisms underlying the pathological states of sleep-wake rhythm is therefore confined to the behavioral level. Therefore, a microscopic level model of sleep-wake rhythm is needed .
The reciprocal interaction model which McCarley and Hobson [4] proposed initiated modeling studies of ultradian rhythm of REM/NREM alternation. Their model is based on the concept that a prey-predator-like interaction between cholinergic and monoaminergic neurons in the brainstem induces the ultradian rhythm, and their later work extended the prey-predator model to the limit cycle model [47, 48]. However, since then knowledge of the neural mechanism regulating sleep and wake states is continuously being updated.
Overview of Recent Neurophysiological Knowledge
Examples of such updates include findings of the involvement of neurons in the ventrolateral preoptic hypothalamic area (VLPO), the extended VLPO (VLPOe), and the median preoptic nucleus (MnPN) in the regulation of NREM as well as REM [8, 9, 49]. The interactions of these neuron groups with those regulating wakefulness and REM in the perifornical/posterior hypothalamic nuclei and the brainstem have been suggested to underlie the physiological mechanisms for maintenance and alternation of sleep and wakefulness [50, 51]. In addition, possible involvement of the glutamatergic and GABAergic neuron groups in the brainstem adds new aspects to regulatory mechanisms of REM [52–54]. So to speak, more light has been shed on glutamatergic neurons in sublaterodorsal nucleus (SLD) in rats (peri-LCα in cats) as an essential physiological substrate of regulatory mechanism of REM [5, 52, 54]. As the recent glutamatergic-GABAergic stories are mainly based on the c-fos studies, Sakai’s studies [5] provide a part of their evidence in terms of neuronal activities [52, 54]. Nevertheless, they have not yet reached a unified story. There are some discrepancies in involvement of forebrain structure in REM regulation and possible contribution of GABAergic inputs toward formation of REM-off activities [5, 52, 54]. Mathematical models could contribute to providing an integrative view to neural regulatory mechanism of sleep-wake rhythm.
Flip-Flop Models of Sleep Regulatory Mechanism
Thus recently accumulated knowledge has prompted sleep researchers to update the prey-predator framework of REM-NREM regulation so that it involves GABAergic systems [55–57]. For the switching mechanisms between wakefulness and sleep as well as NREM and REM, conceptual “double flip-flop” models have recently been proposed [50, 58–60], where the flip-flop is a metaphor of mechanism that each of possible two states is alternatively activated (Fig. 57.2).
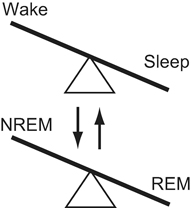
Fig. 57.2
Double flip-flop regime underlying regulation of sleep-wake states. Mutual interactions between them are likely
Although its implementations are different from each other, the flip-flop concept is shared in the recently proposed models [19–22]. Tamakawa et al. proposed one of starting mathematical models based on the recent findings of VLPO and orexinergic neurons, which has been updated so far [19, 22, 61]. Their current model consists of quartet neuron groups: (1) sleep-active preoptic/anterior hypothalamic neurons (S-R group); (2) wake-active hypothalamic and brainstem neurons exhibiting the highest rate of discharge during wakefulness and the lowest rate of discharge during paradoxical sleep or REM (WA group); (3) brainstem and extended VLPO (VLPOe) neurons exhibiting the highest rate of discharge during REM (REM group); and (4) basal forebrain, hypothalamic, and brainstem neurons exhibiting a higher rate of discharge during both wakefulness and REM than during NREM (W-R group). This model can be regarded as a “multi-flip-flop” model in which a flip occurs by accumulation and dissipation of two kinds of sleep-promoting substances, SS1 and SS2: SS1 is accumulated during wakefulness, activates S-R neurons, and is dissipated during sleep. SS2 is accumulated during wakefulness and NREM , activates VLPOe neurons and is dissipated during REM. The reduced minimal configuration of their model is shown in Fig. 57.3. One of novel features of their model is involvement of cholinergic W-R neuron group, which is assumed to contribute toward induction of wakefulness as well as REM by selectively mediating autocatalytic activation with the WA or REM neurons. Through activity of this neuron group, the wakefulness-sleep flip-flop and the REM-NREM flip-flop interact with each other in the model. The model successfully reproduces the normal sleep-wake patterns and those under pathological states [22, 61]. In addition, Phillips and Robinson implemented a flip-flop between arousal system and sleep-promoting system, which consists of the reciprocal interaction between monoaminergic and cholinergic neuron groups involving the mutual inhibition with VLPO neurons [21]. Their model provides a framework accommodating sophisticated dynamical analysis of sleep-wake rhythm and its pathological state such as narcolepsy. The flip-flop mechanism is further investigated by simulating neurophysiological effects exerted by microinjections on sleep-wake rhythms [20], where the model is constructed by referring to the recent knowledge including VLPO sleep promoting GABAergic neurons, brainstem arousal monoaminergic neurons, and brainstem cholinergic REM-on neurons.
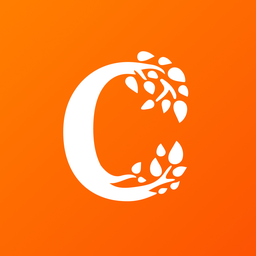
Full access? Get Clinical Tree
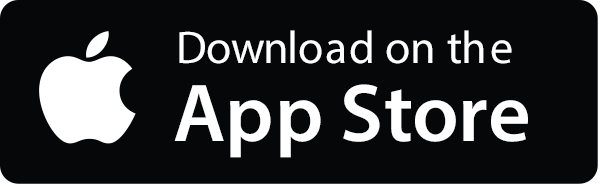
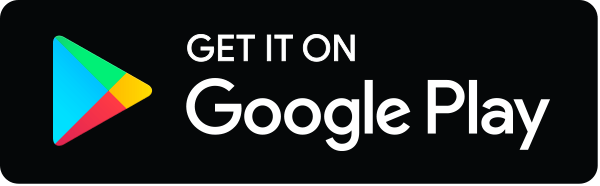