Patient-reported symptom assessments
Penn Spasm Frequency Scale
Spinal cord injury spasticity evaluation tool (SCI-SET)
Patient-reported impact of spasticity measure (PRISM)
Clinical assessments of tone
Ashworth and modified Ashworth
Resistance to passive movement (REPAS)
Pendulum test
Tardieu
Clinical assessments of spastic reflexes
Spinal cord assessment tool for spastic reflexes (SCATS)
Deep tendon and cutaneous reflexes (e.g., Babinski, crossed adduction)
Electrophysiological testing
Reflex testing (assessment of hyperreflexia, reflex modulation)
Poly-electromyography (assessment of dyssynergia)
Patient-reported assessments
No matter how objective or quantitative the spasticity assessment, its value is lessened if it does not correlate with a patient’s own perceptions. The spinal cord injury spasticity evaluation tool (SCI-SET) uses a seven-point Likert scale for each of 34 questions to elicit a person with SCI’s opinion of the positive or negative impacts made by various elements of spasticity [1]. The Penn Spasm Frequency Scale and patient-reported impact of spasticity measure (PRISM) are other self-assessments relating to how spasticity actually affects an individual’s daily life [45, 46]. These types of patient-oriented tools should complement any examiner-based assessment of spasticity.
Ashworth and modified Ashworth Scale (mAS)
This is the most widely used assessment in both clinical practice and research settings [47]. While a subject is supine, the examiner subjectively judges the tone of each examined joint through its range of motion, assigning a score on a five- (unmodified) or six-point (modified) scale between 0 and 4:
(0) No increase in muscle tone
(1) Slight increase in muscle tone, manifested by a catch and release or by minimal resistance at the end of the range of motion when the affected part(s) is moved in flexion or extension
(1+) Slight increase in muscle tone, manifested by a catch, followed by minimal resistance throughout the remainder (less than half) of the ROM
(2) More marked increase in muscle tone through most of the ROM, but affected part(s) easily moved
(3) Considerable increase in muscle tone, passive movement difficult
(4) Affected part(s) rigid in flexion or extension
Any joint can be scored, a feature that makes the mAS flexible yet problematic when different joints are assessed by different examiners among different patients on different days. Furthermore, its ordinal scoring range is not linear, and the 1+ score introduces an extra step into quantifying scores across different patients in research studies. Perhaps most importantly, the Ashworth assesses only one component (passive range of motion) of the spastic condition. Thus, despite its universal use, the Ashworth scale’s inherent subjectivity, unreliability, and limited scope make its nearly universal use inadequate [44, 48–50]. The Tardieu Scale imposes a longer examination time on both patient and clinician, requires greater clinician expertise, and has similar drawbacks that affect interrater reliability [51]. Some of these issues with subjective clinician assessments were addressed by defining the most informative set of joint evaluations across different subjects using the original five-point unmodified Ashworth scale [52].
Pendulum test
This test assesses static tone in the knee joint in a seated subject after release of the leg from an extended position [53, 54]. Though it may be more objective than the mAS, it requires a goniometer for accurate joint angle measurement and use of a rather esoteric formula based on those angles to calculate a spasticity index [55]. These features effectively prevent the pendulum test from any hope of widespread clinical use.
Spinal Cord Assessment Tool for Spasticity (SCATS)
This lower extremity assessment in the supine subject gauges polysynaptic reflex responses to provocative maneuvers such as clonus in response to rapid dorsiflexion of the ankle, toe and leg flexor response to a standardized noxious stimulus to the sole of the foot, and leg extensor response to simultaneous extension of the hip and knee [56]. These maneuvers are adapted from traditional neurological examination techniques but scored in standardized fashion. In theory, SCATS encompasses both a more comprehensive (yet still clinically practical) evaluation and a more objective scoring system than the mAS. The delayed plantar response is another pathological clinical reflex that is not technically scored during the SCATS. This involves plantar flexion of the big toe 0.5–1 s after deep noxious plantar stimulation. The presence of the delayed plantar response indicates more severe injury and a worse prognosis [6, 57].
Electrophysiological spasticity assessments
The routine use of electrophysiological tools to characterize spasticity in the clinical setting is untenable due to costs associated with equipment requirements, technical expertise needed, and time for testing. As research tools, however, these methods are the most direct assessment of the neurophysiology underlying the altered function of the injured nervous system after SCI. As it developed a formalized list of clinical measures for spasticity after SCI (under neurological outcomes), the NINDS Common Data Elements initiative (https://commondataelements.ninds.nih.gov/SCI.aspx) also developed a list of electrodiagnostic testing procedures to neurophysiologically characterize signal conduction and processing in the injured spinal cord. Some of these address aspects of spasticity.
Poly-electromyography
This method studies motor control across groups of muscles and is mostly focused on the pattern of muscle activation for standardized voluntary movements or responses to stereotyped sensory input. One such methodology, long studied but only rarely applied, is the Brain Motor Control Assessment (BMCA) [58–62]. The most helpful feature of such an analysis is the quantitative ability to compare the pattern of muscle activity in injury to that for the same motor task in uninjured individuals. In terms of spasticity, such poly-electromyographic analysis can characterize and quantify the extent of dyssynergia seen under specific circumstances. It can also detect the presence of electromyographic activity when there should be none, probably the electrodiagnostic equivalent of resting hypertonia.
Reflex testing
Reflex or late responses are an established component of peripheral nerve electrodiagnostic testing, but have not been as commonly used in the context of clinical SCI or in measuring spasticity after SCI. As such, the NINDS CDE electrodiagnostic study group did not find a consensus use of testing reflexes, their conditioning, or their modulation under different circumstances in clinical study application. Nevertheless, SCI researchers regularly study Hoffman (H) reflexes [63], posterior root muscle reflexes (PRMRs) [64], tibial reflexes [65], and others to better understand how SCI changes spinal reflexes to generate hyperreflexia and pathological responses.
13.3 Interventions
The spastic state is not exclusively detrimental. Some individuals may exploit spasticity to improve ambulation or mobility [1]. Therefore, the clinician must not assume the goal of eliminating all signs of spasticity. First and foremost, the clinician must listen to the patient to cooperatively set treatment goals and priorities. One patient may prioritize pain relief and improved sleep; another may insist on optimal mobility and refuse any intervention that could interfere with weight bearing; another may desire to fit into a hand orthotic device [66–68]. As with treating many other medical problems, interventions should be titrated. Begin with the least invasive, non-pharmacological treatments, and then range as necessary toward more systemic and invasive options. It should be noted that most of the non-pharmacological treatments do not show definitive benefit when subjected to systematic review; however, this may indicate the difficulty in standardizing the delivery and dosage of physical interventions in formal clinical rehabilitation trials.
13.3.1 Physical Measures
Passive stretching
If a muscle is tight, stretch it. This simple maxim forms the foundation for physical interventions against spasticity. Passive range of motion exercises, either applied by the clinician or ideally self-applied by the affected individual, may reduce hypertonia by directly relieving stiffened intramuscular connective tissue, as well as by inducing accommodation of hyperexcitable muscle spindles and gamma motoneurons [69–72]. The most difficult aspect of passive range of motion exercises is performing them for enough time and repetitions to have a lasting effect. Even “intensive” physical therapy for the typical 2–5 h per week does not make up for the relative muscle inactivity present for the remainder of the 168 h every week. Various types of muscle and joint splints are directed at filling in this inevitable time gap, but when stretching is subjected to systematic review, the overall benefit remains difficult to prove [71–73].
Assisted movements
Assisted upright weight bearing, most often using a standing frame, is commonly used both in the clinic and at home in an effort to achieve multiple goals aside from relieving spasticity – orthostatic tolerance to upright posture, relief of pressure from susceptible skin areas, anti-osteoporotic effects of gravity, and subjective benefits to self-esteem. Assisted standing should also simultaneously stretch and strengthen triceps surae muscles and Achilles tendons. Because of these multiple potential benefits and the relative simplicity and safety, standing frames remain a commonly used intervention despite lack of overwhelming evidence [74, 75].
More active assisted movements are intensively studied for potential benefit on motor control, not just spasticity per se. This includes a range of therapist-guided and robotic-assisted options for functional upper and lower extremity movements (see chapters 22 and 23). For example, body weight-supported treadmill training is directed at lumbar locomotor central pattern generator (CPG) circuits, with the goal of improving CPG coordination and ambulation [76–82]. With more extensive experience in the stroke population, robotic-assisted hand and arm therapy is directed at improving functional reach and grasp motions [83–87]. Both types of therapy may reduce spasticity by replacing aberrant hyperexcitable sensory circuit firing with more functionally appropriate afferent signaling, leading to less muscle spasm and co-contraction [88, 89]. These assisted, pattern-based interventions are thought to induce beneficial neuroplasticity through task-specific learning [41, 90]. It should be noted that to date, the benefits of activity-based therapy have been greater for those individuals with clinically motor incomplete SCI [91, 92]. However, optimism should be retained for those with clinically complete SCI, as combinations of physically assisted therapy with drugs and electromagnetic stimulation may synergize to improve movement in the future even after severe SCI.
Other Physical Measures
A variety of other physical measures are used by therapists to deal with hypertonic muscles, spasms, or unwanted movements in response to sensory stimuli. These include heat, ice, vibration, massage, biofeedback, and relaxation techniques. Small studies have used either focal or whole-body vibration at 50–100 Hz in populations of SCI and stroke patients [93–95].
13.3.2 Drugs
Pharmacological approaches to treating spasticity are regularly reviewed [96–98], so instead this chapter will discuss these agents from the perspective of their impact on the broader issue of motor control after SCI. Compared to physical and other non-pharmacological approaches, drug studies are much easier to standardize in terms of dosing, scheduling, and administration; pharmaceutical companies offer an enormous amount of financial and human capital to back drug studies that cannot be matched by smaller device firms and granting agencies to back non-pharmacological studies; and once at steady state, drugs can exert their influence all 168 h of every week, rather than the handful of hours (at most) that are usually devoted to nondrug interventions. On the other hand, drugs have disadvantages that include more potential for side effects and drug-drug interactions, never mind the general neural inhibition mediated by many spasticity drugs (sedating effects).
The tissues targeted by different anti-spasticity medications vary. While it is not the muscles, the neuromuscular junctions, nor the peripheral nerves that are the site of pathology in SCI, they are often selected for pharmaceutical intervention, in part because they are more accessible than the CNS, and the functional effect can be similar. In some ways, however, this is adding insult to injury, further weakening a damaged nervous system rather than addressing the problem at the site of pathology. Dantrolene, botulinum toxin, and phenol injections weaken muscles, block neuromuscular junctions, and preclude peripheral nerve signal conduction, respectively, and thereby weaken spastic muscles. While decreasing the effects of abnormal motor output, however, they also diminish the capacity for more physiologically appropriate motor production and thereby theoretically limit recovery. We feel these are best used either as add-on medications when others directed at CNS circuitry have failed or when botulinum toxin injections are targeted at a specific, overly active muscle or sub-portion of a muscle that is reliably perturbing a specific motor function (discovered perhaps through poly-electromyography applied during specific tasks).
Pharmacological agents that address CNS neural circuitry are more directed toward reimposing inhibition at the site of pathophysiology in SCI. Nevertheless, the use of these medications may resemble using a sledgehammer as a fly swatter, as none of the medications are precise enough. It is nearly impossible to use these agents to impact just the altered neural circuitry leading to hyperreflexia or hypertonia or disordered coordination between motoneuron pools (dyssynergias) without also decreasing physiologically appropriate motor output. Whether we are talking about pre- or postsynaptic inhibition or action at GABA A, GABA B, alpha, 5HT, or dopamine receptors, drugs like benzodiazepines, baclofen, tizanidine, cyproheptadine, or dopamine agonists (respectively) should be used at minimally effective doses for the individual patient to best improve functional status. There is clinical experience, if not randomized clinical trials, to suggest that combinations of these drugs may be as or more effective than high doses of just a single line of pharmacological attack.
To better deliver drug to the site of impaired neural circuitry, baclofen can be given intrathecally instead of orally [99]. Intrathecal delivery trials can be pursued to ascertain clinical effect prior to the permanent implantation of an intrathecal pump, but it seems this course of action is often pursued before a full exploration of the effect of oral medication combination therapy. While intrathecal pumps can be very effective, and appeal to many with difficult to control spasticity, there is a rare but real risk of life-threatening baclofen withdrawal syndrome due to pump failure, disconnection, or poor management. All should be aware that pruritus in the absence of a rash in an intrathecal baclofen user is withdrawal until proven otherwise and that oral replacement is often insufficient in these cases. If it is not feasible to reestablish intrathecal delivery quickly (risk of infection with pump pocket abscess, for instance), intravenous benzodiazepine therapy may be necessary to stabilize the baclofen withdrawal patient.
One advantage to intrathecal delivery of baclofen is that it can be combined with intrathecal delivery of medications for neuropathic pain such as opioids, clonidine, and local anesthetics (“-caines”) [100, 101]. This raises the issue of the interaction between pain and spasticity. Many patients use changes in their spasticity as an indication of other noxious stimuli or pathology they cannot consciously feel. Certainly, both muscle afferents and cutaneous nociceptive afferents can activate involuntary motor output in SCI, so conjunctively treating pain and spasticity in parallel makes sense. In patients taking gabapentin or pregabalin for chronic pain, they often report improvements in spasticity. When necessary, a urinary tract infection associated with worsened spasms should be treated not only with antibiotics but also with a short course of increased pain medication along with a short-course increase in anti-spasticity medication dosing.
13.3.3 Electromagnetic Stimulation
Some electromagnetic stimulation paradigms are hailed as panaceas for pain, spasticity, and motor skills, but as with most non-pharmacological interventions, the evidence lags the hype. This is partly due to the infinite number of stimulus parameters that may be applied, leading to difficulty in comparing results from numerous small studies using different paradigms. We will briefly touch on several popular (or promising) electromagnetic interventions:
Transcutaneous electrical nerve stimulation (TENS)
TENS units were developed to treat pain based on gate control theory [102]. However, once the FDA approved TENS unit marketing for home use, TENS use became widespread for weakness and spasticity as well. A typical TENS session comprises ~30–60 min of ~100 Hz stimulation at an intensity above sensory but below motor threshold [103]. The unit is usually placed over peripheral nerves serving the affected muscles but sometimes is placed directly over the affected muscles. When subjected to formal systematic review in multiple sclerosis, TENS failed to show benefit for spasticity [104]. However, many smaller studies in SCI and stroke have shown positive results [103, 105–108]. The mechanism of its effect in spasticity is not well understood [102, 109]. Regardless, given its safety profile and relatively affordable price, TENS will remain a popular non-pharmacological treatment option for the foreseeable future.
Functional electrical stimulation (FES)
This methodology has been developed for decades to enhance volitional muscle control to achieve functional tasks of daily living by externally stimulating the appropriate nerves in the appropriate sequence [110]. Widely used FES-cycling systems allow individuals with SCI to more easily obtain cardiovascular workouts [110, 111]. However, FES recruits motor units in an unnatural sequence that results in clumsy, easily fatigued muscle responses [111, 112] (see chapter 24). This easy fatigability remains a fortuitous side effect in the context of spasticity, as fatigued muscles are less likely to spasm [7, 113–116].
At a more fundamental level though, by targeting peripheral nerves, FES bypasses all of the intrinsic circuitry that the central nervous system has evolved to carry out movements in the most efficient and coordinated manner. Successful modeling has not yet been achieved to recapitulate all of the feedback and feedforward loops that regulate groups of synergistic agonists and antagonists, that stabilize proximal muscle groups while distal muscles execute fine tasks, and that adjust movements in response to incoming sensory information. Whether human technology can outdo millions of years of evolution within the next several decades remains to be seen.
Transcranial magnetic stimulation (TMS)
As opposed to stimulating directly over affected muscles, repetitive TMS (rTMS) targets cerebral origins of altered motor control. At pulse frequencies of 1–5 Hz, rTMS negatively modulates underlying cortical excitability, whereas at 5 Hz or greater, rTMS positively modulates underlying cortical excitability [117]. At excitatory frequencies, rTMS has been shown to reduce spasticity in SCI, multiple sclerosis, and cerebral palsy populations, presumably through increasing descending inhibitory drive to inhibitory interneuronal circuits [118–123]. Interestingly, although clinical spasticity and reciprocal inhibition improve in response to rTMS, H-reflex amplitudes do not consistently normalize in these studies [119, 121]. Conversely, inhibitory 1 Hz rTMS was shown to increase H-reflex amplitude, further supporting a model in which temporarily reducing descending cortical drive increases spinal cord hyperexcitability [118, 122]. Thus, multiple sessions of excitatory rTMS hold promise not only for reducing spasticity in SCI but also for improving motor control once appropriate combinations with other forms of activity-based therapies are found.
Direct current stimulation (DCS)
This technique uses almost imperceptibly low current intensity, but it has had an outsized impact in numerous disease and performance-enhancing contexts – spasticity included [124–128]. The low-intensity current is far below action potential threshold, but given with the anode over the targeted site (either transcranial or transspinal), DCS seems to modulate neuronal membrane excitability in a serotonin- and NMDA-dependent manner [129–131]. However, several major gaps in mechanistic understanding persist: there is no technique to directly map how the low-energy current distributes within the body or to determine how individual variations in injury characteristics affect that distribution. Furthermore, the continuous nature of DCS makes it difficult if not impossible to elucidate timing-dependent synaptic changes. Therefore, although DCS has shown therapeutic benefit in spasticity and other contexts, its underlying mechanisms are quite likely to remain a black box.
Spinal cord stimulation
An increasing number of groups are studying electromagnetic stimulation over the cord, which in our opinion is the most rational target for modulating spasticity. The first indication that spinal cord stimulation could relieve spasticity came after the advent of epidural spinal cord stimulators that had been designed for pain relief via gate control theory [132]. Epidural spinal cord stimulators were then fortuitously noted to relieve spasticity symptoms in multiple sclerosis patients [133, 134]. Since that time, expanding work has shown benefits not just for pain and spasticity but for improved volitional control as well [135–139]. Noninvasive spinal stimulation, delivered either via phasic or continuous direct current, has shown similar promise without the surgical risk or expense, making it a more attractive candidate for widespread application [53, 139, 140].
Both invasive and noninvasive spinal cord stimulation appear to activate local afferent circuits that trans-synaptically amplify signals transmitted along spared descending fibers. The cited studies have applied stimulation at various sites along the cord, in patterns ranging from constant direct current to pulsatile frequencies between 10 and 1500 Hz. When targeted caudally to a lesion to combat lower extremity spasticity, frequencies of 50–100 Hz applied to the upper lumbar region are most effective, whereas frequencies of 20–60 Hz most effectively engage the locomotor CPG [139].
It remains to be seen whether a “sweet spot” can be found in which stimulation simultaneously reduces spasticity and enhances volitional motor control over multiple muscles or whether stimulation patterns will need to be dynamically adjusted depending on the task being performed. However, its ability to facilitate proper functioning of excitatory and inhibitory circuits simultaneously in their naturally intertwined milieu imparts spinal cord stimulation with extraordinary potential to improve all aspects of motor control after CNS injury.
13.4 Conclusion and Needs in the Field
Despite intense study for centuries, nervous system function and malfunction remain very poorly understood. Spasticity is no exception. The field has basic needs: better clinical and physiological assessment, better electrophysiological understanding, and, of course, better treatment. These are all inextricably linked.
The fact that SCI characteristics vary among human individuals makes things more complicated on the surface, but this variation also holds the key to better understanding. Potential correlations between spasticity phenotype and injury structure and physiology remain largely unstudied. Development and rigorous analysis of more relevant animal models could provide invaluable insight into the links between structure, function, and spasticity that would not be possible in human patients.
In humans, quantitative electrophysiological studies need to be melded with quantitative magnetic resonance imaging and clinical characterization of each individual’s SCI phenotype. Obviously, this will require standardized, quantitative clinical spasticity assessments with properly curated metadata stored in public data repositories with consensus de-identification methods and public usage protocols.
Once the assessment and data repository infrastructure is in place, then “big data” algorithms should be able to exponentially improve our understanding of how SCI phenotype correlates with SCI structure and physiology. Incidentally, there has been (and likely always will be) much more study devoted to spasticity after stroke and multiple sclerosis than after SCI. Further studies need to specifically attend to unique features of SCI spasticity, and why they differ from features of other types of spasticity.
Thus, spasticity needs to be rebranded – the perception of spasticity as nervous system hyperactivity needs to be replaced with a more physiologically appropriate perception as an altered state of motor control. With this mindset, we are optimistic that future interventions providing individually customized combinations of patterned, circuit-specific activation should be able to simultaneously improve both spasticity and motor control.
References
1.
Adams MM, Ginis KAM, Hicks AL (2007) The spinal cord injury spasticity evaluation tool: development and evaluation. Arch Phys Med Rehabil 88:1185–1192. doi:10.1016/j.apmr.2007.06.012 PubMed
2.
Sköld C, Levi R, Seiger A (1999) Spasticity after traumatic spinal cord injury: nature, severity, and location. Arch Phys Med Rehabil 80:1548–1557PubMed
3.
Westerkam D, Saunders LL, Krause JS (2011) Association of spasticity and life satisfaction after spinal cord injury. Spinal Cord 49:990–994. doi:10.1038/sc.2011.49 PubMedPubMedCentral
4.
Ditunno JF, Little JW, Tessler A, Burns AS (2004) Spinal shock revisited: a four-phase model. Spinal Cord 42:383–395. doi:10.1038/sj.sc.3101603 PubMed
5.
Hiersemenzel LP, Curt A, Dietz V (2000) From spinal shock to spasticity: neuronal adaptations to a spinal cord injury. Neurology 54:1574–1582PubMed
6.
Ko HY, Ditunno JF, Graziani V, Little JW (1999) The pattern of reflex recovery during spinal shock. Spinal Cord 37:402–409PubMed
7.
D’Amico JM, Condliffe EG, Martins KJB et al (2014) Recovery of neuronal and network excitability after spinal cord injury and implications for spasticity. Front Integr Neurosci 8:36. doi:10.3389/fnint.2014.00036 PubMedPubMedCentral
8.
Leis AA, Kronenberg MF, Stĕtkárová I et al (1996) Spinal motoneuron excitability after acute spinal cord injury in humans. Neurology 47:231–237PubMed
9.
Weaver RA, LANDAU WM, HIGGINS JF (1963) Fusimotor function. II. Evidence of fusimotor depression in human spinal shock. Arch Neurol 9:127–132PubMed
10.
Little JW, Ditunno JF Jr, Stiens SA, Harris RM (1999) Incomplete spinal cord injury: neuronal mechanisms of motor recovery and hyperreflexia. Arch Phys Med Rehabil 80:587–599, S0003-9993(99)90204-6 [pii]PubMed
11.
Tansey KE, McKay WB, Kakulas BA (2012) Restorative neurology: consideration of the new anatomy and physiology of the injured nervous system. Clin Neurol Neurosurg 114:436–440. doi:10.1016/j.clineuro.2012.01.010 PubMed
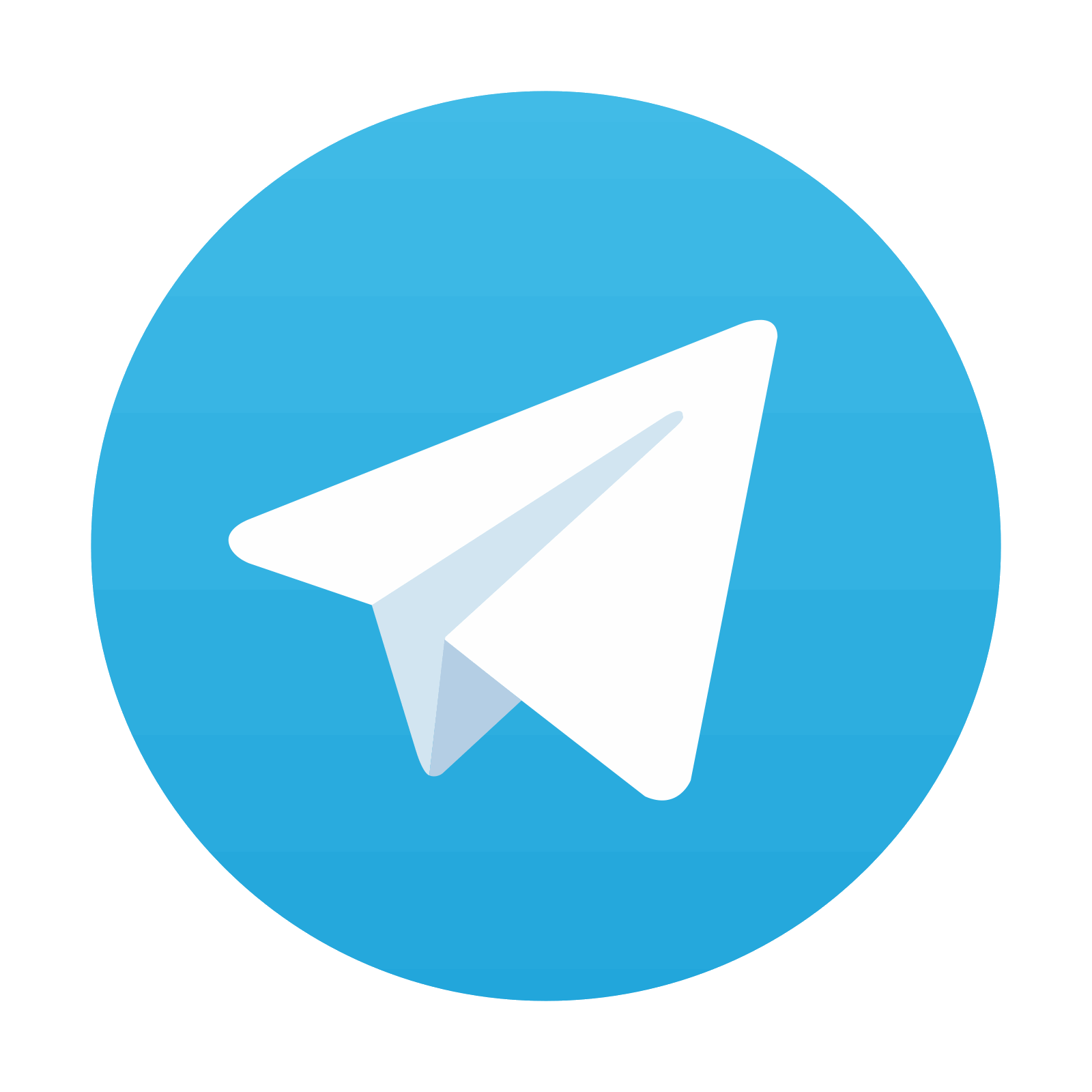
Stay updated, free articles. Join our Telegram channel
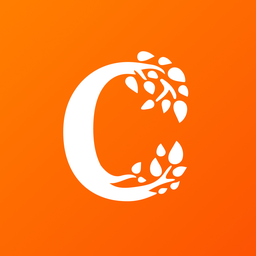
Full access? Get Clinical Tree
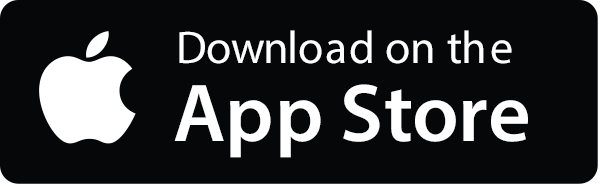
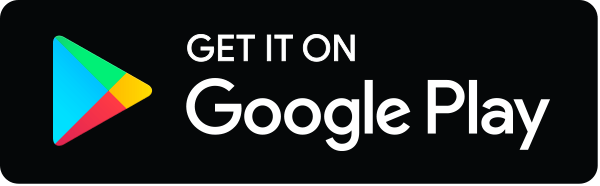