The development of functional mapping techniques gives neurosurgeons many options for preoperative planning. Integrating functional and anatomic data can inform patient selection and surgical planning and makes functional mapping more accessible than when only invasive studies were available. However, the applications of functional mapping to neurosurgical patients are still evolving. Functional imaging remains complex and requires an understanding of the underlying physiologic and imaging characteristics. Neurosurgeons must be accustomed to interpreting highly processed data. Successful implementation of functional image-guided procedures requires efficient interactions between neurosurgeon, neurologist, radiologist, neuropsychologist, and others, but promises to enhance the care of patients.
To carry out effective and safe neurosurgical interventions for patients with brain lesions, precise information about the unique structural and functional anatomy of that patient is essential. Eloquent cortical areas cannot be recognized solely by identifying known anatomic landmarks even in nonlesional brains. In patients with mass lesions or longstanding epilepsy, physical distortion or compensatory reorganization can further alter normal relationships. To select patients for surgery, plan the operative approach, and successfully execute the surgical goal, detailed information regarding the individual structural and functional anatomy and the relationship to the lesion is potentially useful. Thus several brain mapping methods that have been developed for neuroscientific brain mapping efforts have been adapted to serve neurosurgical considerations. There are fundamental differences in how these methods are applied to neurosurgical patients and problems. Unlike group studies for neuroscience in which inferences are made about a population based on a sample, neurosurgical planning requires single-subject–specific information, spatial precision, adequate accommodation for impaired task performance, prioritization of sensitivity more than specificity, and robustness in the face of mass lesions. In the last decade, much progress has been made toward fulfilling these needs using several techniques.
This article discusses the particular opportunities and challenges associated with brain mapping as it applies to patient selection, presurgical planning, and intraoperative guidance. The principal methods and their relative strengths and weaknesses are discussed. Approaches for mapping cortical motor, sensory, and language areas are reviewed, as well as the associated white matter connections. Some practical and technical considerations are also discussed, including patient-specific concerns; issues related to data acquisition, analysis, and interpretation; and visualization considerations for planning and intraoperative use. In addition, the specific role of brain mapping in patients with specific pathologic processes including brain tumors, vascular malformations, and medically refractory epilepsy is considered.
Brain mapping techniques
Direct cortical stimulation (DCS) and the intracarotid amytal test (IAT) or Wada test are often considered the gold standard procedures for functional mapping and language lateralization, respectively. Their status as gold standard is based on long experience with these procedures as well as the way in which these procedures localize eloquent brain. Both procedures involve deactivation, in which a brain region, either localized in the case of DCS, or nearly hemispheric in the case of IAT, is taken off line, and the patient’s neurologic function tested. In this way, the procedures mimic what deficits might be expected after resection. Although these procedures have proved to be effective, they are highly invasive mapping techniques that carry significant risk of morbidity, and which necessitate active patient cooperation during testing. Functionally impaired patients, or those with an altered level of consciousness, may find it difficult to perform the tasks, thus decreasing the value and broad applicability of these procedures. DCS of the gray matter within the depth of the sulci is also usually not readily accessible. Furthermore, DCS is conducted either within the surgical procedure or a short time before it, leaving little time for effective surgical planning or for the contemplation of alternative therapeutic strategies.
Thus, the recent development of less invasive mapping techniques has provided an appealing alternative or adjunct for many neurosurgeons. Most of these techniques map the brain by recruiting the functionality of a particular brain region through a behavioral task (paradigm) and measuring consequent brain changes. For instance, during functional magnetic resonance imaging (fMRI) or magnetoencephalography (MEG), patients are asked to perform a task, such as a language, visual, or movement paradigm, while changes in blood flow, metabolism, or electric activity in activated brain regions is measured. Although this general approach can show all functional brain regions that are involved in the execution of a particular task, it cannot differentiate between brain regions that are essential for execution of the task and those that are merely playing a supportive role. However, inhibition (blocking) methods, like DCS or IAT, temporarily disrupt a specific brain region from functioning, thereby testing for an inducible neurologic deficit. Transcranial magnetic stimulation (TMS) may act as an inhibition method (eg, for language mapping), but more commonly is used for neurosurgical mapping as a direct activator (eg, for motor mapping). This method stimulates a given brain region directly, rather than by engaging the subject in a behavioral paradigm, and can thus depict the causal relationship of the brain tissue in question to the task execution. However, this approach also has limitations. Even direct mapping of the primary motor cortex with TMS may not show all of the areas involved in motor performance. Inhibition methods may fail to show sufficiency. For instance, the disruption of face motor pathways, or even of the temporalis muscle, by TMS could cause speech arrest without interfering with language function per se. In addition, diffusion tensor imaging (DTI) although not a functional mapping study, is able to provide information on the location and trajectory of white matter tracts that may inform interpretation of functional brain areas. Thus, it is critical that the neurosurgeon understands the strengths and weaknesses of each approach to maximize the benefit from presurgical mapping.
Whether the mapping technique is observational, such as positron emission tomography (PET), MEG, or fMRI, an inhibition technique such as TMS, or an advanced structural map such as DTI, these brain mapping modalities are rapidly acquiring an expanded clinical role in the surgical planning phase. Each technique is based on different physiologic properties, thus providing different types of functional maps. Furthermore, each imaging modality is characterized by a specific set of advantages and disadvantages related to spatial and temporal resolution, to the degree of invasiveness, and to the costs of implementation. Combining different functional mapping methods, although resource and time intensive, may provide optimal information by offsetting the strengths of one technique against the weaknesses of another or by integrating functional and structural information. With all of these methods, when used for surgical planning, consideration must be given to sensitivity and specificity: to not miss an eloquent brain region, it is particularly important to avoid false negatives, thus maximizing sensitivity, sometimes at the expense of specificity.
Understanding the physiologic and technical underpinnings of each mapping method is particularly important to use them most effectively for clinical decision making. For example, PET detects the relative position of radioactively labeled compounds within the patient’s body, and can thus provide a wide range of functional and physiologic data. PET is emerging as a tool to guide the location of biopsies to avoid sampling error leading to undergrading of tumors that may have heterogeneous histologic characteristics. PET is also frequently used to show areas of interictal hypometabolism associated with epileptogenic foci. Areas being developed include radiotracers targeted to specific receptors, which may eventually guide functional neurosurgery. However, the signal-to-noise ratio (SNR) and temporal resolution of PET are both poor, and the spatial resolution in best-case scenarios is considered to be only moderate. DTI can quantify the magnitude and direction of water diffusion in brain tissue, and is thus able to show the location and trajectory of white matter tracts. However, DTI is vulnerable to signal loss artifacts from air spaces, and provides little information about the functional status of the depicted white matter tract. As routinely deployed, DTI cannot reliably resolve crossing white bundles and can also have difficulty showing tracts with high curvature. However, by combining DTI with fMRI, it may be possible to make inferences about which tracts are important to preserve, based on their physical continuity with cortical areas of interest. MEG noninvasively maps brain activity by measuring changes in the local magnetic fields that accompany neuronal activity. MEG is most commonly used in the presurgical evaluation of epileptic patients. In contrast with PET scanning and DTI, the temporal resolution of MEG is excellent: on the order of 1 millisecond. However, the spatial resolution is variable and dependent on the model used to approximate the source of the signal, and is susceptible to environmental magnetic noise. TMS is an emerging mapping technique that induces neuronal changes by delivering magnetic fields at the scalp that pass unimpeded through the scalp and skull and are able to stimulate the cortex in a manner analogous to DCS. In TMS, electrical activity in a functional brain region may be either stimulated or inhibited depending on the mode of stimulus delivery via a magnetic field change delivered to the scalp. This technique could provide a substantial advantage to the neurosurgeon because it is the only noninvasive modality that can block neuronal function. However, TMS requires dedicated equipment and personnel, has not yet been reliably able to map language function, and may be contraindicated in patients with seizures.
fMRI as a noninvasive presurgical mapping technique
Presently, the most commonly used functional mapping procedure for surgical planning is fMRI, a functional mapping technique that measures the relative changes in oxygenated and deoxygenated hemoglobin, and thus blood flow, as a surrogate for neuronal activity. Being completely noninvasive and able to be deployed on many clinical magnetic resonance imaging (MRI) scanners, its clinical role has rapidly expanded. The physiologic information shown by fMRI can be acquired with, and coregistered to, corresponding structural images in a straightforward way. The safety and relative ease with which fMRI can be acquired allows its use not only for planning optimal surgical strategy but also to guide the decision whether to perform surgery and for patient counseling regarding risk. Furthermore, the registration of preoperatively acquired fMRI data into a neuronavigational guidance system can help guide the deployment of intraoperative electrocortical stimulation.
What can be expected from functional mapping in presurgical planning?
The indications for the use of functional brain mapping continue to evolve as technology develops and becomes more broadly applied. In 1999, Lee and colleagues retrospectively evaluated the effect of fMRI on the treatment plans of 46 patients scheduled for neurosurgical resection of either a tumor or epileptogenic foci. The group determined that fMRI contributed substantially in 3 key areas: (1) allowing the neurosurgeons to assess the risk of inducing a neurologic deficit, allowing them to evaluate the feasibility of the resection; (2) providing substantial neurosurgical guidance by directing the placement of the bone flap or of subdural grids for mapping or electroencephalogram (EEG) recording; and (3) helping to identify patients who required further evaluation through invasive mapping techniques. Overall, the study concluded that fMRI studies were used in at least 1 of the 3 clinical steps in 89% of patients with tumors and 91% of patients with epilepsy. Petrella and colleagues conducted a similar prospective study, analyzing the role of sensorimotor fMRI localization on 39 neuro-oncological patients being evaluated for potential neurosurgical resection. The investigators reported that fMRI altered patient treatment approaches in 49% of patients and allowed the neurosurgeons to further maximize the extent of tumor resection in 45%.
The effect of PET scanning on the neurosurgical treatment of pediatric patients with brain gliomas has also been investigated. The study group concluded that the addition of PET scans into the neuronavigational guidance system substantially improved the diagnostic yield of glioma biopsies, and optimized designated surgical trajectories. In addition, the number of trials needed to reach gliomas situated in remote locations, such as those near the pineal gland, was significantly less when PET scanning was used. The ability of MEG scanning to predict the epileptogenic foci in patients with refractory seizures has been examined and found to be nearly as helpful as intracranial EEG in correctly predicting the epileptogenic foci (MEG, 57%, intracranial video-EEG, 62%).
fMRI as a noninvasive presurgical mapping technique
Presently, the most commonly used functional mapping procedure for surgical planning is fMRI, a functional mapping technique that measures the relative changes in oxygenated and deoxygenated hemoglobin, and thus blood flow, as a surrogate for neuronal activity. Being completely noninvasive and able to be deployed on many clinical magnetic resonance imaging (MRI) scanners, its clinical role has rapidly expanded. The physiologic information shown by fMRI can be acquired with, and coregistered to, corresponding structural images in a straightforward way. The safety and relative ease with which fMRI can be acquired allows its use not only for planning optimal surgical strategy but also to guide the decision whether to perform surgery and for patient counseling regarding risk. Furthermore, the registration of preoperatively acquired fMRI data into a neuronavigational guidance system can help guide the deployment of intraoperative electrocortical stimulation.
What can be expected from functional mapping in presurgical planning?
The indications for the use of functional brain mapping continue to evolve as technology develops and becomes more broadly applied. In 1999, Lee and colleagues retrospectively evaluated the effect of fMRI on the treatment plans of 46 patients scheduled for neurosurgical resection of either a tumor or epileptogenic foci. The group determined that fMRI contributed substantially in 3 key areas: (1) allowing the neurosurgeons to assess the risk of inducing a neurologic deficit, allowing them to evaluate the feasibility of the resection; (2) providing substantial neurosurgical guidance by directing the placement of the bone flap or of subdural grids for mapping or electroencephalogram (EEG) recording; and (3) helping to identify patients who required further evaluation through invasive mapping techniques. Overall, the study concluded that fMRI studies were used in at least 1 of the 3 clinical steps in 89% of patients with tumors and 91% of patients with epilepsy. Petrella and colleagues conducted a similar prospective study, analyzing the role of sensorimotor fMRI localization on 39 neuro-oncological patients being evaluated for potential neurosurgical resection. The investigators reported that fMRI altered patient treatment approaches in 49% of patients and allowed the neurosurgeons to further maximize the extent of tumor resection in 45%.
The effect of PET scanning on the neurosurgical treatment of pediatric patients with brain gliomas has also been investigated. The study group concluded that the addition of PET scans into the neuronavigational guidance system substantially improved the diagnostic yield of glioma biopsies, and optimized designated surgical trajectories. In addition, the number of trials needed to reach gliomas situated in remote locations, such as those near the pineal gland, was significantly less when PET scanning was used. The ability of MEG scanning to predict the epileptogenic foci in patients with refractory seizures has been examined and found to be nearly as helpful as intracranial EEG in correctly predicting the epileptogenic foci (MEG, 57%, intracranial video-EEG, 62%).
How valid is functional mapping for surgical planning?
Given the relative newness of the functional mapping methods, there remain numerous issues related to the validity and reliability of the methods in different conditions. One area of note is that several of these methods evolved out of neuroscience efforts, and most publications are related to group results. Functional mapping for neurosurgical planning must inform about individual subjects, and the cost for erroneous results may lead to significant morbidity or, at the other extreme, to undertreatment of a lesion that might be surgically addressed. Thus, ongoing validation of the brain mapping approaches against each other and against the gold standard methods of Wada testing and intraoperative DCS mapping is particularly important. A lot of work has been done examining agreement between mapping approaches and, increasingly, examining effects on patient care.
Although fMRI offers substantial advantages to the neurosurgeon, its use in clinical decision making should allow for several technical difficulties. Functional MRI does not assess neural activation directly, but measures the associated variations in the cerebral blood flow using blood oxygen level–dependent (BOLD) contrast on T2*-weighted images. Thus, an intact autoregulatory response is required for proper signal interpretation. Pathologic processes in the brain that disrupt the neural hemodynamic coupling may alter the concordance between neural activity and the cerebral blood flow. For example, the signal can be disrupted by the mass effect of a lesion, or large cerebral veins can induce susceptibilities that can also distort the BOLD signal interpretation. These issues can diminish the sensitivity and specificity of functional brain mapping. Furthermore, fMRI acquisition and analysis as yet have no accepted standard procedure. There is no standardized battery of behavioral paradigms, and determination of the significant threshold of activation is a subjective process. The statistical analysis of the data is diverse; different statistical approaches can be followed in the data interpretation. Furthermore, fMRI only depicts the topography of the functional cortical areas; it does not provide information about the white matter connections, which are equally important in maintaining the proper neuronal functionality of the patient. Because of these limitations, data interpretation and clinical decisions need to be made with caution.
Several studies have shown a higher incidence of neurologic deficits in patients who underwent resections that were within 0.5 to 2 cm of functional cortex when fMRI alone was used to map functional regions, as opposed to using DCS. For instance, Mueller and colleagues used fMRI to map the functional cortex and showed that postoperative neurologic deficits occurred in 0%, 33%, and 50% of cases in which the resection margins were beyond 2 cm of the eloquent cortex, within 1 to 2 cm of the eloquent cortex, and less than 1 cm from the eloquent cortex, respectively. Although these studies advocated intraoperative DCS to map functional cortex, when the spatial distance between the lesion and the functional cortex was less than or equal to 2 cm; it should also be emphasized that these early studies concluded that fMRI was a safe and reliable mapping tool that carried great promise for the future.
Statistical analysis in clinical implementations of fMRI
Activation in fMRI is dependent on the statistical threshold and a multitude of other factors. When the statistical threshold is lowered, the area of activation increases, and when the statistical threshold is raised, the area of activation decreases ( Fig. 1 ). Thus, fMRI does not measure absolute values, and therefore cannot be used to infer absolute spatial distances. Although a specific description of the statistical methods implemented to analyze fMRI data is beyond the scope of this article, the color-coded maps presented for fMRI studies depict statistical likelihoods, usually as T or Z scores. In addition, variation exists in the magnitude, shape, and location of a signal across different individuals performing an identical task in controlled conditions. The reason for this varied fluctuation has not been precisely determined. Furthermore, there is also intrasubject variability in the signal generation that could be influenced by numerous physiologic and psychological factors, such as caffeine ingestion, sleep deprivation, level of attention, and fatigue. For neuroscience applications, group analysis merges the data across different subjects, and random-effects analysis helps to diminish the effect of individual variability. However, for surgical planning, capturing individual variability is the object of the exercise, but avoiding, or at least being aware of, potential artifacts is critical for accurate inferences.
Spatial precision: smoothing and the inverse solution
Spatial smoothing is a common step implemented during the analysis of fMRI and PET datasets to improve SNR and because it is a prerequisite for standard statistical analysis using the general linear model. For neuroscientific studies of groups, smoothing is also used to reduce the effect of functional and anatomic variability within and between individual subjects. Smoothing is usually implemented as a convolution of spatial data with a Gaussian kernel. Neighboring voxels within a predefined spatial extension are averaged and smoothed into a single voxel value. This processing step optimizes the signal strength and enhances the blob appearance of active brain regions on functional maps. However, this added benefit comes at the undesirable cost (particularly for surgical planning) of decreasing the spatial resolution of the functional maps. The merging of activation centers can blur and shift them distorting their anatomic depiction on the functional maps. In addition, smoothing creates partial volume artifacts along the edge of the brain, because high-intensity brain tissue voxels are averaged with low-intensity voxels outside the brain. Maisog and Chmielowska addressed this issue, and successfully described a method to correct the artifacts along the edges of the brain. Other approaches using data-driven analysis strategies, such as independent component analysis (ICA), have also been proposed that do not require spatial smoothing.
Localization of function (or signal sources) based on the observation of recorded signals at the scalp (ie, MEG and EEG), requires assumptions about a model to which the data are fitted, which is known as the inverse problem of estimating the model parameters based on the data. In the case of MEG and EEG, the inverse solution is ill posed, thus requiring that some assumptions be included to solve the problem. In clinical MEG, a model that is frequently used is the single equivalent current dipole. This model assumes that the generator of the measured signal originates from a single source. In practice, this approximation may result in solutions that do not accurately localize the true source or sources of the signal. Other approaches have been proposed, including distributed solutions, but to date there is no consensus on how best to localize the signals.
In practice, it is important with any mapping method that a measure of the spatial uncertainty be presented with the data. For MEG results, generally only dipoles with goodness of fit of 70% or more are displayed. The written report should also be inspected for a description of how many of the dipoles were discarded, and so forth. For fMRI, the unthresholded or dynamically thresholded statistical maps can provide some information about the location of the boundary of an activation, although there remains no established method of showing the degree of uncertainty. In general, information about acquisition voxel size and the size of the smoothing kernel, if applied, is not given in processed clinical fMRI. Discussion between the individuals acquiring, processing, and interpreting the studies is important in understanding the limits of the data.
Shortcomings and considerations of particular techniques
The validity of any functional mapping data interpretation is dependent on numerous preconditions: physiologic assumptions are accurate, adequate SNR, patient cooperation during the data acquisition, and the methods of analysis. Different techniques will be best for different situations and this article describes some technique-specific considerations.
fMRI
Functional MRI indirectly assesses the level of neuronal activation by measuring the relative changes in the concentrations of oxy- and deoxyhemoglobin present in the microvasculature. However, several studies have suggested that signal distortion can also be generated from larger draining veins not directly involved in the activation process (in the so-called vein effect), a phenomenon that can substantially decrease the precision of the spatial localization. Abduljalil and colleagues suggested that the use of spin echo sequences can reduce this distortion from the macrovasculature downstream of the activated area. However, spin echo sequences are less sensitive to magnetic susceptibility effects (which are the basis of the BOLD signal), and thus require longer scanning acquisition times, and cover smaller areas.
Head movement during fMRI acquisition severely degrades data quality. Not only can excessive head motion result in supplemental false activation, it can also decrease or even obscure real activation by altering the signal time course so that the synchronous magnetic resonance (MR) signal from the task paradigm is concealed. This effect can be caused by both abrupt and gradual head movements ( Fig. 2 ). Krings and colleagues further showed that motion-related signal artifacts in paretic patients occurred in a significantly higher ratio than in patients without a paresis (72% vs 29%). These findings are believed usually to be related to compensatory movement efforts recruiting uninvolved, usually proximal, musculature. We have found that patients have more head motion than healthy controls. In general, if the patient’s maximal displacement is more than 2 mm, we repeat the acquisition once to see whether better motion parameters can be achieved. If not, then the data are not deemed interpretable.
Bold fMRI reliance on susceptibility imaging can be problematic. For example, susceptibility artifacts are created at the junction of air and tissue interfaces, such as in the vicinity of the tympanomastoid air cells of the middle fossa, and from the nasal and sinus airspaces in the orbitofrontal cortex, causing geometric distortions and dropouts in signal intensity. Furthermore, susceptibility artifacts can also be evident in postsurgical patients because of the presence of surgical clips, titanium plates, metal dust from a skull drill, or prior blood products. The presence of these paramagnetic objects can create significant susceptibility that can mask the signal generated from the surrounding neural cortex. Thus constant caution is required in the fMRI data interpretation of postsurgical patients. The customary habit of overlaying the functional brain maps on T1-weighted conventional images can also be troublesome, because the artifacts may no longer be discernible.
MEG
MEG scanners and the shielded room required to house them are expensive, which limits broad clinical applicability outside a few centers. Currently, the cost of installing a MEG scanner can be more than $2 million; MEG scanners also require dedicated personnel. A further drawback of MEG scanners is their extreme susceptibility to surrounding magnetic fields, such as the magnetic noise of the hospital, and even the Earth’s own magnetic field.
The clinical applicability of MEG is further hindered by the large spatial distance that separates the source of the signal and the MEG detectors; decreasing both the spatial resolution and the accuracy of signal source localization. Interpreting MEG signals is also problematic whenever multiple sources in the brain become active simultaneously. Then, an infinite number of ways could be used to interpret the signal. For instance Liu and colleagues showed that several signals that were generated from several sources that were 1 to 3 cm apart were erroneously portrayed as a single superimposed signal on MEG scanners.
PET
PET scanners are characterized by a low spatial and temporal resolution. Furthermore, the SNR of PET scanners is weaker than the SNR of other noninvasive mapping techniques such as fMRI. The temporal resolution is especially limited because a substantial temporal delay exists between the imaging phase of the procedure and the visualization phase of the metabolically active foci that discern the neuronal changes. Moreover, PET is a invasive procedure that invokes the administration of a radioactively labeled tracer. Thus, certain patient populations, such as children, are excluded from PET imaging. Similarly to MEG scanning, PET imaging is also expensive, and also requires dedicated personnel. The equipment of PET scanners is also highly priced. Furthermore, a complete PET scanning platform would require a cyclotron to generate radioactive tracers. Similarly to fMRI, PET scanning is also an imaging modality that is hemodynamically based. Thus, it is also a technique that is susceptible to signal distortions from uncoupling issues. PET scanning is also an observational technique and can thus not differentiate functional areas that are essential for the task execution from those areas that are only supportive to the task execution.
DTI
DTI is presently the only method that can allow the visualization of white matter tracts in vivo. However, several technical limitations still hinder its complete clinical applicability for surgical planning. For instance, three-dimensional (3D) tractrography is generally seeded over the whole brain, creating a large mass of fibers that obscure visualization. Several approaches have been developed to aid in the selective visualization of tracts, including seeding from specific anatomic or functional landmarks. Nevertheless, for tracts in close proximity to a brain lesion, it may be difficult to tell whether the tract is running within the lesion or just beyond it. In a recent study, Golby and colleagues addressed these issues by developing an interactive software tool that can show all white bundle tracts within a specific distance from the tumor boundary, and allows the clinician to instantaneously redefine the spatial distance required, enabling the clinician to view all the tract layers around a specific brain lesion. In addition, the software also included the ability to place seedpoints in regions of interests, which also allow the clinician to visualize all the tracts passing through that particular location. Because DTI depends on the fractional anisotropy of white matter to define areas of tightly bundled fibers, anything that lowers the anisotropy, such as tumor infiltration or edema, may falsely obscure the presence of preserved tracts. Also, like some of the functional mapping methods, the visualization of DTI results depends on setting several thresholds such as the fractional anisotropy (FA) and curvature-stopping thresholds. Thus, there is a subjective element to which tracts may be visualized. Furthermore, DTI does not provide any information that relates to the interactions between white bundle tracts and functional cortical regions. Without a priori knowledge acquired from functional mapping such as fMRI, it can be hard to determine the reciprocal interactions between white bundle tracts and the functional cortex. Furthermore, DTI cannot identify eloquent structures within the depicted white bundle tracts, nor can it recognize tracts that are necessary for task execution.
Practical and technical considerations
Hardware
All brain mapping techniques require dedicated hardware. For many centers, fMRI will require the least capital investment, because fMRI can be performed on most modern clinical scanners and technical personnel can be trained in additional skills. DTI can also be acquired in addition to any routine clinical studies. In the past 10 years, the most commonly available magnetic field strength was 1.5 T. Today, 3 T field strength is widely being recognized as the optimal strength for most clinical applications. As a notable advantage, the SNR is substantially higher. The signal at 3 T is expected to be 4 times stronger than at 1.5 T, but the noise also increases twofold, and thus only results in a net 2 times factor increase in the SNR. However, this higher resolution in the imaging scans can have important clinical implications, and provides neurosurgeons with a substantial advantage during the surgical planning phase. At 3 T, spatial resolution can be improved, allowing more precise mapping. Advances in coil design have now made multichannel (8- or 16-channel, or even 32-channel) head coils available. The increase in SNR from the multichannel coils can be used to shorten acquisition times or to allow for smaller voxels. Shortening the data acquisition phase can decrease the occurrence of motion artifacts because it is easier for patients to tolerate and makes it more feasible to acquire both structural and functional images in 1 sitting. Overall, designing fMRI scanning paradigms involves making tradeoffs about acquisition time, voxel size, and scan coverage. Although higher field strength and multichannel coils can provide more SNR, the issues of how to effectively maximize acquisitions remain important.
Compared with fMRI, PET and MEG require significant investment. Both methods require expensive scanners, dedicated and specially shielded space, and specially trained personnel. Institutions that already have these suites set up, either for existing clinical applications or research efforts, are likely the sites where these types of studies will be performed in the near future. In addition, functional PET scanning will require the support of nuclear chemists and a cyclotron to maximize the potential. TMS setups are much less expensive to acquire, but have limited penetration in clinical sites to date.
Software
Software plays a critical role in the acquisition and analysis of data from all the brain mapping methods. In addition to the basic processing software, there are frequently issues of separate software systems that need to work together. The most common format for processed images remains Digital Imaging and Communications in Imaging (DICOM; Medical Imaging and Technology Alliance, Arlington, VA, USA), although other formats such as Neuroimaging Informatics Technology Initiative (NIfTI; jointly sponsored by National Institute of Neurological Diseases and Stroke and National Institute of Mental Health) are also being developed. It is particularly important to have personnel with good working knowledge of the software. In going from the acquisition phase on the imaging device to a processing computer and then to a display or navigation system, there are numerous steps in which potential inconsistencies can be introduced. An audit trail of data integrity is particularly important when multiple platforms are used. Some groups have developed fully automated pipelines for data analysis and display that can help to ensure quality control and streamline workflows.
The most commonly used software packages for fMRI and PET have been Brain Voyager and Statistical Parametric Mapping (SPM; Wellcome Department of Neurology, London, United Kingdom). Although these software packages are able to import DICOM images, perform two-dimensional (2D) and 3D statistical data analysis, and analyze single- and multiple-subject data sets, these applications were mainly designed for research use, and they were not tailored for the clinical interpretation of single-subject data or for use by clinicians. With the expanding role of fMRI in the clinical domain, multiple manufactures have begun to develop software tailored for clinical applications. Applications have been designed for use on the major MRI scanner platforms as well as by third-party vendors. Most packages include both stimulus delivery for controlling behavioral paradigms and analysis and visualization software. These software packages, unlike the earlier research packages designed for neuroscience research, do not generally focus on strategies for analyzing multiple-subject data such as normalization and random effects statistics, nor is there the degree of customization generally available in research platforms. Instead, because they have been designed for clinical applications, the focus is on single-subject acquisition, standardization of procedures, ease of use, and regulatory compliance. Several applications have the ability to monitor and display the activation maps with only minimal delay, thus providing direct visualization of statistical maps as the data are being acquired. A substantial advantage of this feature is its ability to monitor the quality of the data at the time of the study. Thus, if poor fMRI maps are being generated, the study can be stopped and any complicating factors, such as stimulus display problems, misunderstanding of task instructions, excessive head motion, and so forth, can be addressed before the patient leaves the suite. The postacquisition packages can perform a semiautomatic in-depth analysis of the datasets that are based in part on the older research software. Thresholding can be performed at the time of acquisition or, ideally, can be dynamically changed by the interpreting clinician. After analysis, data may be represented as contoured or segmented structures on the 2D images or as 3D colored functional maps. In turn, the data can be viewed in any plane, or may be projected onto a reconstruction of the individual’s cortical surface, thereby providing a useful surgical planning tool to the neurosurgeon ( Fig. 3 ).
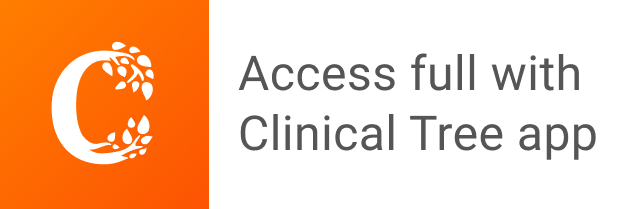