Fig. 11.1
Somatotopic organization of the spinal cord. Within white matter: green illustrates sensory and red motor fiber tracts. Within gray matter: red depicts anterior horn alpha-motoneurons. Somatotopy-specific clinical and neurophysiologic measurements are illustrated in the text boxes. SSEPs somatosensory evoked potentials, dSSEPs dermatomal somatosensory evoked potentials, QST quantitative sensory testing, EPTs electrical perception thresholds, EPP electrical pain perception, MEPs motor evoked potentials, ENG electroneurography, EMG electromyography, LT light touch, PP pinprick, CHEPs contact heat evoked potentials, LEPs laser evoked potentials
During the acute setting, clinical questions mainly focus on elementary motor (e.g., muscle strength), sensory (e.g., sensation, discrimination), and autonomic (e.g., bladder emptying) functions and the early prediction of outcome [37, 177]. In this context, neurophysiology is commonly requested not only in order to objectify the clinical exam but also to offer an even higher precision than the clinical exam alone, i.e., providing a higher sensitivity and specificity with particular respect to the completeness of the injury. Despite such understandable clinical needs, not neurophysiology but the standardized neurologic examination still represents the mainstay and “gold standard” for a meaningful clinical classification, grading, and, based on this, the prediction of outcomes after SCI [42, 69, 92, 157, 159, 170–172, 180] (see chapter 4). Compared to a structured neurologic examination according to the International Standards for Neurological Classification of Spinal Cord Injury (ISNCSCI) [4], no single or combined neurophysiological technique provides a better classification or prediction accuracy with respect to global or specific (e.g., ambulation) outcomes after SCI [150, 168]. Clinical classifications acutely after the SCI, i.e., >72 h after injury [15, 62, 69, 80], can sufficiently predict long-term neurological and functional outcomes, enabling physicians to tailor rehabilitation plans accordingly. Frequently, traumatic SCI is associated with concomitant injuries [75, 121, 124]. Particularly for patients with concomitant brain injury, clinical classification can be inaccurate [121]. For those situations or in, e.g., patients under sedation, neurophysiology can be a useful tool to detect neurological dysfunction as was demonstrated by, e.g., fractionated somatosensory evoked potential (SSEP) recordings [85].
With respect to the monitoring and objectification of neurological and functional recovery after SCI, conventional MEP and SSEP recordings seem to be of limited value [43, 53, 173]. However, a large longitudinal dataset suggested that clinical recovery (motor scores and ambulation) was paralleled by an increase in MEP amplitudes to the tibialis anterior muscle [135]. Furthermore, surface electromyography (EMG) recordings correlate with motor recovery [111, 123]. Dermatomal SSEPs (dSSEPs) in combination with, e.g., electrical perception threshold testing are currently under clinical investigation and might serve as suitable techniques in order to monitor segmental spontaneous recovery and potential treatment effects in the future [58, 96, 99].
Later, in subacute and chronic stages after SCI, not only elementary but also complex functional interactions of neurological systems (e.g., sensorimotor interaction during ambulation, locomotor training) are of major importance to patients and clinicians. Neurophysiology might help here, to understand basic pathophysiological mechanisms and guide locomotor rehabilitation after SCI [52, 86, 93]. In this respect the analysis of reflex studies or patterns of muscle activation might help to guide and individualize neurorehabilitation after SCI [160].
Technically, neurophysiologists focus on amenable and recordable functionality of long spinal white matter tracts (spinal pathways) after SCI specifically on (1) descending motor corticospinal tracts, (2) ascending sensory dorsal column tracts, and (3) ascending sensory spinothalamic tracts. Corresponding fiber tract-specific ISNCSCI assessments are (1) motor scores, (2) light touch sensation, and (3) pinprick sensation. Motor scores, however, do not exclusively measure the functionality of spinal motor pathways. In fact, the total central and peripheral corticomuscular pathway influences motor scores. Similarly, sensory scores are influenced by parts of the peripheral and central nervous system and not only by the spinal cord lesion. In clinical routine, several neurophysiological techniques exist to measure (1) corticomuscular (transcranial magnetic stimulation (TMS)), (2) somatosensory (somatosensory evoked potentials (SSEP)), and (3) spinothalamic (laser evoked potentials (LEPs), contact heat evoked potentials (CHEPs)) pathways. In addition to these global (i.e., not spinal pathway specific) neurophysiological assessments, it is technically possible to identify afflicted peripheral or central parts of the nervous system by the proper selection of stimulation-recording montages. Neurophysiological techniques and their clinical value in a SCI-specific context are discussed in this chapter. As pointed out for the clinical exam, neurophysiology is intended to detect evidence for motor/sensory/autonomic incompleteness with a particular focus on subjectively unbiased recordable evidence.
11.2 Motor Evoked Potentials (MEPs)
MEPs are muscle responses evoked by transcranial electrical (TES) or magnetic stimulation (TMS) of cerebral motor neurons in order to analyze the total corticomuscular pathway [5–7, 28, 72, 125]. Within the motor cortex, TMS directly and indirectly (transsynaptically) stimulates a subpopulation, mainly large-diameter, fast-conducting corticospinal fibers, which make direct monosynaptic contacts with spinal alpha-motoneurons. After stimulation of the motor cortex, muscular responses can be recorded as compound muscle action potentials over muscles of clinical interest. TMS has been widely adopted in clinical routine because the cortical stimuli are painless and the method is clinically helpful in a number of neurological disorders [50]. With a combination of both, TMS and a peripheral motor nerve conduction study to measure the peripheral motor conduction time (PMCT) calculations of the central motor conduction time (CMCT) are possible. PMCT can be approximated by two neurophysiological methods:
Magnetic spinal nerve root stimulation
F-wave recording: PMCT = (F + M − 1)/2 (this method is only feasible for distal muscles)
CMCT is then calculated as the difference of the corticomuscular latency and the PMCT.
MEPs are routinely analyzed with respect to potential latencies in milliseconds and amplitudes in millivolts. Another parameter is the resting motor threshold, measured in % of the stimulators maximum output, at which at least 50 % of muscular responses with amplitudes of at least 50 μV occur. Compared to rest, a voluntary contraction of the target muscle substantially enhances its response to magnetic brain stimulation and reduces latencies (facilitation) [81]. Moreover, the variability of latencies and amplitudes is much less during facilitation. In general, latencies are less variable compared to amplitudes [28, 127]. In clinical routine, therefore, latencies during facilitation are the preferred and most accurate readout parameter in MEP recordings.
In incomplete SCI, MEPs to muscles below the lesion level usually have prolonged latencies, reduced amplitudes, increased resting motor thresholds, and impaired facilitation [14, 19, 45, 46, 70].
There is some evidence that in upper extremities despite a complete loss of voluntary muscle function, MEPs can be elicited, which might argue for a clinical complete, however subclinically incomplete, lesion [70]. Another MEP approach to uncover incompleteness is the attempt to modulate spinal reflexes below the neurological level (e.g., subthreshold flexor reflex H-reflex stimuli) by brain stimulation [76, 77, 175]. For an analysis of discomplete lesions with other techniques than MEP recording, see Sect. 11.6 [153]. Furthermore, after SCI the cortical representation of muscles changes which can be mapped by TMS [109, 163].
Patients with absent leg muscle function do usually have a loss of MEPs to the leg muscles in the very acute phase (within 6 h post-injury, spinal shock phase) [118] and also in later phases [19, 26, 29, 135]. For the prediction of 6-week motor recovery an ultra-early MEP investigation within 6 h after injury appears to be unsuitable, since no correlation with later muscle function was found. Only in already clinically apparent, voluntarily contracting muscles, MEPs could be evoked [118]. In later stages however, e.g., 1 month post-injury, patients with preserved normal MEP latencies to both the quadriceps femoris and tibialis anterior muscles do achieve a full ambulatory function defined as no or little disturbance in walking (for the definition of ambulatory function, see [31, 39, 88]).
Over a 1-year course after SCI, MEP latencies in incomplete SCI were usually stable and thereby unsuitable for monitoring of spontaneous recovery. However, in a subgroup of patients with initially (within 15 days) recordable but delayed MEPs to the tibialis anterior muscle, latencies shortened (38.56 ± 3.77 vs. 35.4 ± 6.08 ms) and amplitudes increased (0.68 ± 0.63 vs. 1.32 ± 0.92 mV) over the 1-year course, and these changes correlated with improvements of lower extremity motor scores (13.2 ± 7.3 vs. 23.2 ± 2.6) and walking function as measured by the 10 m walking test (0.55 ± 0.66 vs. 0.86 ± 0.99 m/s) [135]. Therefore, in certain subgroups of incomplete SCI, MEP to the tibialis anterior muscle might be of value as a subclinical measure of spontaneous recovery or even as additional outcome measure for treatment effects. However, it remains uncertain if MEP changes add value to the examination of motor scores or walking function alone.
In incomplete SCI, MEPs to the abductor digiti minimi muscle in the acute phase were shown to predict hand function at around 6 months post-injury [39]. In this study, hand function was defined on an ordinal scale from 0 to 2 (0 = no function; 1 = active wrist extension with passive grasp via tenodesis effect; 2 = active grasping including intrinsic hand muscles). A loss of MEPs to the abductor digiti minimi muscles measured at a median of 25 days post-injury was associated with a failure to recover active grasping in 90 % of patients. If this prediction quality is better or independent from motor score analysis alone remains uncertain.
11.3 Somatosensory Evoked Potentials (SSEPs)
It is long known (around the 1950s) that afferent volleys to the cerebral cortex can be recorded after electrical stimulation of the median or ulnar nerves at the wrist [47, 48]. SSEPs can be used to evaluate both somatosensory pathways of the peripheral and the central (i.e., spinal, subcortical, cortical level) nervous system. The examined somatosensory part of the nervous system depends on the selection of (1) the stimulation and (2) the recording sites. Depending on the neurophysiological equipment (number of recording channels) multiple recording sites can be analyzed. Such fractionated analyses are also feasible in SCI patients with a supraspinally isolated spinal cord and even during the spinal shock phase [151]. SSEPs can be evoked from almost any nerve. In clinical routine, however, standardized stimulation-recording montages for tibial, median, ulnar, or pudendal nerves are usually applied [32, 120]. The somatosensory evoked potentials are usually analyzed with respect to early potential component latencies in milliseconds and amplitudes in microvolts. In relation to a standardized clinical exam, tibial nerve SSEPs correlate well with light touch scores, i.e., the dorsal column function, and poorly with pain perception, i.e., spinothalamic function [10]. Higher stimulation intensities lead to more reliable cortical tibial nerve SSEPs after SCI [9].
Already in the acute stage, within 1–2 weeks after SCI, SSEP recording is feasible and can be clinically meaningful [27, 94, 179]. An absence of cortically evoked tibial nerve SSEP in the acute setting correlates with the clinical completeness of SCI. Furthermore, abolished cortical SSEP from any stimulation site in the acute stage are associated with poor neurological recovery. Several investigators studied the capacity of SSEP recordings as early predictors for ambulatory function [31, 36, 110, 142, 161]. Whereas agreement exists for the predictive value of absent cortical SSEP for poor neurological outcome, the value of preserved but pathologic SSEPs for good outcome is less clear. In one study the preservation or an early (within 1 week) return of tibial nerve SSEPs was associated with a favorable prognosis with respect to ambulatory function after a median follow-up of 12 months [142]. In another study a positive correlation of preserved acute-stage (within 2 weeks) cortical tibial or pudendal nerve SSEPs with 6-month ambulation was observed (ambulation defined on an ordinal scale: 0 = no, 1 = therapeutic, 2 = functional, 3 = full ambulation; for a detailed definition, see [31, 88]). This correlation was true for both, SSEPs measured on a continuous scale (latencies/amplitudes) and on an ordinal scale [36]. Ordinal scaling might be preferred over continuous scaling since clinical interpretation is more straightforward (1 = absent SSEP, 2 = delayed latency and reduced amplitude, 3 = delayed latency and normal amplitude, 4 = normal latency and reduced amplitude, 5 = normal latency and normal amplitude; see Fig. 11.2) [94]. Full ambulation defined as no or little disturbance in walking was achieved in 83 % of patients with preserved SSEP of types 4 and 5, whereas in patients of SSEP types 2–3 only 10 % and in patients of type 1 no patient achieved full ambulation. In a further analysis to predict 6-month ambulation measured by walking index for spinal cord injury (WISCI II) and 6 min walk test (6MWT) in subjects with motor incomplete SCI (ASIA Impairment Scale (AIS) C or D), lower extremity motor scores measured within 16–40 days after injury were the strongest predictor for ambulation 6 months after injury. In tetraparetic subjects, a combination of lower extremity motor scores and cortical tibial nerve SSEPs (if not symmetrical, then of the worse leg) compared to lower motor extremity scores alone improved prediction accuracy slightly (90 versus 92 % correct classifications) [181]. If such a small improvement of prediction accuracy is clinically meaningful remains to be established.


Fig. 11.2
Principle of the SSEP classification according to Kovindha and Mahachai [94] using tibial nerve SSEP as an example. Latency in milliseconds on the horizontal axis. Amplitude in μVolts on the vertical axis. Dashed vertical lines indicate the latencies of P40. SSEPs are classified on an ordinal scale where lower values are worse. (1) Abolished SSEP, (2) prolonged latencies and reduced amplitudes, (3) prolonged latencies and normal amplitudes, (4) normal latencies and reduced amplitudes, and (5) normal latencies and amplitudes. SSEPs with normal latencies are depicted with a blue background. SSEPs with prolonged latencies or abolished potentials are depicted with a red background. Such categorization was, e.g., applied in the work of [36] in order to predict outcome
It was stated that despite neurological improvement, cortical SSEP latencies in incomplete SCI do not significantly change over a 1-year course after SCI compared to the initial exam during the acute stage. And it was concluded that this finding might argue for neural plasticity as the more likely underlying repair mechanism compared to axonal regeneration/remyelination [43]. In a further detailed analysis of the 1-year time course (within 15 days vs. 1 year) of cortical tibial nerve SSEPs, however, a certain subgroup of incomplete patients with recordable cortical tibial nerve SSEPs showed shortening of latencies (52.1 ± 1.0 vs. 48.9 ± 0.1 ms) and increase of amplitudes (0.8 ± 0.2 vs. 1.3 ± 0.2 μV). Moreover, these changes were paralleled by an improvement of light touch scores (1.3 ± 0.1 vs. 1.5 ± 0.1) and walking function as measured by the 10MWT (43.1 ± 8.1 at 1 month vs. 17.7 ± 6.5 s) [156]. Hence, for certain subgroups of incomplete patients, cortical tibial nerve SSEPs might be useful for the monitoring of spontaneous recovery or for the analysis of treatment effects. However, it remains an unanswered question, whether tibial nerve SSEP analysis adds value to the analysis of light touch scores or walking function alone.
Ordinal-scaled (five categories as described above for tibial nerve SSEPs [94]) pathological cortical ulnar nerve SSEPs measured 1 month after cervical SCI are of value as a predictor for active hand function at 6 months after injury (0 = no function, 1 = active wrist extension with passive grasp via tenodesis effect; 2 = active grasping including intrinsic hand muscles; 3 = normal or only mildly disturbed hand function) [35]. More precisely, in patients with ulnar nerve SSEP categories 1–3, i.e., prolongation or loss of ulnar nerve SSEP at 1 month, 96 % remained with an inactive hand function (hand function categories 0–1) at 6 months. The positive predictive value of ulnar nerve SSEPs at 1 month however is quite imprecise. Only 48 % of patients with normal ulnar nerve SSEP latencies developed active or normal hand function. Slightly more accurate as a positive predictor for active or normal hand function performed cortical median nerve SSEPs, as 72 % of normal latency patients developed active or normal hand function. Another detailed study on the longitudinal evolution of cortical ulnar nerve SSEP over a 1-year period after cervical SCI corroborated the predictive value of preserved cortical ulnar nerve SSEPs within 1 month after injury for the prediction of hand function as measured by the SCIM subitem “feeding” [24, 25] at 1 year [104].
With respect to the recovery of urinary bladder function, the predictive values of cortical tibial/pudendal nerve SSEPs were investigated [41]. SSEP recordings were performed on average of 10 days after SCI and were categorized according to Kovindha et al. [94]. As outcome at 6 months, two measures were analyzed: (1) classification according to the urodynamic examination and (2) classification according to the function of the external urethral sphincter (1 = normal voluntary contraction, sensory, and detrusor function; 2 = impaired, i.e., preserved voluntary contraction and disturbed micturition due to impaired sensory and/or detrusor function; 3 = absence of function, i.e., loss of voluntary contraction and impaired sensory/detrusor function). In tetraplegic patients cortical pudendal nerve SSEPs were not clearly associated with bladder function. However, 20 % of cortical pudendal nerve SSEPs were not recordable, and this was interpreted as false-negative results as they were expected to be present. Cortical tibial nerve SSEPs were associated with 6-month outcome of both urodynamic and external urethral sphincter functions. All tetraplegic patients with normal cortical tibial nerve SSEP latencies (categories 4–5) and all paraplegic patients with normal cortical pudendal nerve SSEP latencies (categories 4–5) developed a normal bladder function as measured by the external urethral sphincter classification at 6 months. For SSEP categories 1–3, i.e., prolongation or absence of the SSEPs, the development of a normal bladder function as measured by the external urethral sphincter classification at 6 months was basically null for paraplegics and very unlikely in tetraplegics.
The diagnostic and prognostic value of cortical tibial nerve SSEP recorded at the lumbosacral level (lumbosacral evoked potentials [55, 107]) with particular respect to the differentiation between a hyper-reflexive and acontractile detrusor function is not fully clear yet. Urodynamic examinations are the diagnostic mainstay for this highly relevant and prevalent clinical question [11, 116].
11.4 Nerve Conduction Studies (NCSs) and Electromyography (EMG)
Motor nerve conduction studies of median, ulnar, radial, and peroneal nerves and sensory nerve conduction studies have been introduced into the clinical routine over 60 years ago in the middle of the twentieth century [48, 71, 83]. Even further back (the 1930s) dates the clinical application of needle electromyography [49, 113] with major contributions later on in the 1940s/1950s [16, 17, 102, 103]. Guidelines for a proper conduct of NCS/EMG in the clinical context have been published [13, 23, 158]. Applying transcutaneous bipolar electrical stimulation to peripheral nerves, time-related motor and/or sensory responses can be evoked and recorded. Registration can routinely be performed using skin surface electrodes. For motor nerve conduction studies, the evoked compound muscle action potentials (CMAPs) after supramaximal nerve stimulation are analyzed. For sensory nerve conduction studies, the ortho- or antidromically evoked sensory nerve action potentials (SNAP) are investigated. Latencies and stimulation-recording site distances are used to calculate conduction velocities in meter/s. Potential amplitudes in millivolt (CMAP) and microvolt (SNAP) are used to interpret axonal damage to peripheral nerves. In motor nerve conduction studies, not only the direct M-responses but also late muscle responses like F-waves and H-reflexes can be elicited in order to investigate more proximal parts of the peripheral nerves (i.e., proximal to the utmost amenable proximal simulation site). Using needle EMG, one can determine acute and/or chronic neurogenic changes of innervating nerves of the respective muscles.
In the clinical context of SCI, the mentioned studies can help to detect additional nerve root or peripheral nerve injuries [8, 20, 68]. Moreover, electroneurography (ENG) assessment of peripheral arm nerves in combination with the knowledge of the spinal segmental innervation can help to infer intramedullary alpha-motoneuron damage and hereby predict the recovery of hand function in tetraplegics with lesion levels between C6 and T1 [34]. In this study hand function was defined as (1) “active hand” where the patients were at least able to use two kinds of handgrips (the pulp pinch and the lateral pinch) and (2) “inactive hand” where patients were unable to perform those handgrips. Motor ENG assessments of ulnar and median nerves were graded on an ordinal scale from 1 to 4 (1 = no CMAP (complete Wallerian degeneration); 2 = reduction of CMAP and conduction velocity (severe axonal neuronopathy); 3 = reduction of CMAP an normal conduction velocity (mild axonal neuronopathy); 4 = normal CMAP and conduction velocity). ENG measurements were performed during the first 2 weeks post-injury. Hand function (active hand vs. inactive hand) was analyzed 3 months post-injury. 90 % of hands in the ENG groups 3–4 (mild axonal neuronopathy or normal) recovered active hand function, whereas only 35 % of hands in ENG groups 1–2 (complete Wallerian degeneration or severe axonal neuronopathy) recovered active hand function. All hands of ENG groups 1–2 for both median and ulnar nerve remained inactive.
For caudal spinal cord lesions, ENG might help to discern central (epiconal) and peripheral (conal/cauda equina) nerve damage after Wallerian degeneration and consecutive CMAP reductions of tibial and peroneal nerves. Hereby it was also possible to quantitatively assess the extent of the conus/cauda lesions [144]. Severe axonal lesions at the thoracolumbar level are associated with a poor prognosis for recovery of ambulation [87, 132].
In chronic SCI ENG/EMG studies might help to diagnose secondary nerve disorders like entrapment syndromes (e.g., carpal tunnel syndrome, common peroneal pressure palsy) [131]. Moreover, secondary damage to the peripheral nervous system below the SCI lesion level can be assessed [89, 91, 112, 166].
F-waves are late muscle responses evoked by a supramaximal antidromic electrical stimulus to a peripheral motor nerve, which occur several milliseconds after the M-response [57, 126]. The term F-waves dates back to 1950 when recordings were firstly performed from the small intrinsic muscles of the foot [119]. F-waves are much smaller, usually <5 % in amplitude, compared to the corresponding M-responses. They are believed to be generated by an echoing bioelectrical impulse in axons of alpha-motoneurons. After a distal supramaximal stimulation of a motor nerve, the antidromic conduction wave reactivates a small pool of anterior horn cells once arrived at their axon hillocks within the spinal cord causing an orthodromic conduction wave in a small number of alpha-motoneurons back to the muscles. Absent F-waves indicate a reduced excitability (such as hyperpolarization) of spinal anterior horn motoneurons. There is a clear temporal correlation of abolished F-waves with the duration of spinal shock [38, 82]. Furthermore, F-wave chronodispersion, i.e., the difference between the minimal and maximal F-wave latency, might serve as an indicator for anterior horn excitability with increased chronodispersion in SCI patients with spasticity [164].
H-reflexes are late muscle responses to transcutaneous electrical stimulation of a mixed/motor nerve [18, 67, 84]. The clinically most widely performed H-reflex of the soleus muscle is basically the electrically provoked monosynaptic Achilles tendon tap reflex (stretch reflex). Anatomically, the reflex is organized as a monosynaptic arc of afferent (Ia fibers), spinal segmental, and efferent pathways (segmental reflex pathway). The recorded response is dependent on the stimulus intensity. Submaximal electrical stimuli provoke the response when there is still no M-response. With increasing stimulus intensity, the M-response amplitude increases and the H-reflex amplitude diminishes. Supramaximal stimuli lead to a disappearance of the H-reflex.
H-reflexes can be generated even in spinal shock, a stage early after trauma characterized by a profound depression of spinal reflex activity, when tendon tap reflexes are still absent [51, 114]. The discrepancy between the presence of an H-reflex and the absence of tendon reflexes might best be explained by a reduced excitability of gamma-motoneurons which drive fusimotor activity [82] or the more complete and synchronous volley of afferent input provided with electrical stimulation relative to mechanical stimulation. Compared to flaccid spinal cord injuries, spasticity seems to be associated with increased H-reflex amplitudes [161]. However, in another study on chronic SCI, H-reflex characteristics were not different compared to able-bodied persons [148]. Since intrathecal treatment of spasticity with baclofen reduces H-reflex amplitudes, H-reflex recordings might be helpful in monitoring antispastic treatment effects in patients with SCI [117].
Bulbocavernosus and anal reflex: These reflexes are summarized here, since recording techniques are comparable to CMAP analysis in ENG recordings. Both reflexes are polysynaptic spinal reflexes at the sacral level, which can be provoked by sensory (e.g., tactile/electrical) stimuli to the penis/clitoris and perianal region, respectively.
The reflex arc of the bulbocavernosus reflex consists of sensory afferents of the pudendal nerve, spinal neurons of the sacral segments S2–S4, and motor efferents of the pudendal nerve. Different methods exist to provoke and record the reflex (e.g., mechanical stimulus and visual/tactile recording, mechanical stimulus and electrical recording, electrical stimulus and recording). For an accurate assessment of latencies and amplitudes, however, an electrical stimulus and needle/surface EMG recording montage are required. If side differences are anticipated, a needle approach is necessary [2, 3, 56, 143, 155]. The bulbocavernosus reflex is one of the first recovering spinal reflexes after SCI and can already be elicited in spinal shock. Hence, in the clinical context of acute SCI, the loss of the bulbocavernosus reflex indicates a conus medullaris or cauda equina lesion [130]. Not only in the acute setting but also in later stages after cauda equina lesions the bulbocavernosus reflex remains absent or pathologic [61].
Generally, an abolished bulbocavernosus reflex is associated with a urinary bladder dysfunction of the lower motor neuron type. Despite similarities in the neurological pathway for the activation of the bulbocavernosus and external urethral sphincter muscles [134], exact urodynamic characteristics cannot be predicted by the presence or absence of the bulbocavernosus reflex [115, 152].
The presence of a bulbocavernosus reflex in chronic male SCI patients might be of value for the selection of an ejaculation method (i.e., penile vibratory stimulation versus others like electroejaculation) [12].
The anal reflex [141] can be provoked mechanically or electrically by stimulation of the anal mucosa or the perianal skin. Electrical stimulation paradigms of, e.g., the tibial nerve, which are sufficient to provoke flexor reflexes, can also provoke an anal reflex [133]. Standardized methods for the electrical stimulation and electromyographic recording of this reflex have been described [133]. Such a standardized technique is necessary since mechanical stimulation and visual recording is insufficient to consistently elicit this reflex [1, 128]. Conditional on an intact reflex arc after SCI (i.e., cauda equina and conus medullaris need to be intact), the anal reflex can already be evoked in spinal shock [133, 139]. Hence, the loss of this reflex after SCI is associated with a conus medullaris or cauda equina lesion. The neurological pathways for an activation of the external anal sphincter and the external urethral sphincter seem to be different. Hence, a dysfunction of the external anal sphincter is not necessarily associated with a dysfunction in the external urethral sphincter. Analyses in this respect can only be done sufficiently via simultaneous pelvic floor/sphincter ani EMG during urodynamics [95, 176].
11.5 Autonomic Studies
The sympathetic skin response (SSR) is a measurement of sudomotor induced resistance changes in response to arousal stimuli, which can be, e.g., acoustic or somatosensory [30, 105, 129]. In clinical routine a standardized electrical stimulus to, e.g., the median nerve is utilized. For patients with high cervical SCI who are not able to perceive a median nerve stimulus, a nerve above the level of injury, e.g., the supraorbital nerve, is preferred [21, 178]. With respect to recording, sympathetic outflow to the sudomotor sweat gland of hands, feet, and the perineal region can be analyzed. The SSR gives an indication of how well the connections from the brain to the sympathetic nervous system in the thoracolumbar (Th1-L2) spinal cord are preserved. Depending on the SCI level and completeness, SSRs can be missing completely or partially. In complete cervical SCI, the SSR to hands, feet, and perineum is usually missing, whereas these responses are preserved in lesions below L2 [21, 44, 140]. Absent SSRs have been shown to be associated with autonomic dysreflexia [40, 44].
With respect to the stimulation site (i.e., stimulation below the neurological level), one interesting finding is that even in complete cervical or thoracic lesions (above L1/not cauda equina), plantar SSRs can be evoked after electrical stimulation of the pudendal nerve (approx. spinal segment S3) [138]. Since the lumbar and sacral spinal cord needs to be intact for the SSR to be evoked, these findings might argue for a sympathetic sacrolumbar spinal reflex circuit, which is independent from supraspinal sympathetic outflow. Stimulation of the tibial nerve (approx. spinal segment S1) was insufficient to evoke plantar SSRs. This is in line with the finding that increased intravesical pressures during cystometry lead to skin responses below the lesion in complete SCI patients [136].
Following electrical stimulation of the median nerve, a selective perineal SSR loss (with preservation to hands and feet) is indicative for a thoracolumbar Th10-L2 sympathetic spinal lesion and strongly associated with bladder neck incompetence/dysfunction after SCI [140]. After electrical stimulation of the median nerve or TMS, the absence of hand and feet SSR was associated with both autonomic dysreflexia and detrusor sphincter dyssynergia in cervical SCI. In thoracic SCI SSR absence to hand and feet was associated with detrusor sphincter dyssynergia but not with autonomic dysreflexia [149].
11.6 Other Methods
Routine SSEP and MEP recordings basically represent a global “longitudinal” assessment of spinal white matter tracts. However, sensitivity in order to detect changes at the segmental level is usually poor. Hence, more sensitive but still specific segmental tests are needed. For this purpose, dermatomal SSEPs, analysis of electrical perception threshold (EPT), and electrical pain perception (EPP) are currently under clinical investigation [59, 90, 96, 99, 106, 108, 145, 147, 167, 169]. Moreover, segmental MEP recordings at the thoracic level from the erector spinae muscle might improve outcome assessment in “ISNCSCI-blind” thoracic motor levels [22, 60]. Such segmental approaches might be of particular clinical importance as outcome measures in clinical trials with putatively small treatment effects [73].
With respect to sensory assessment, additional neurophysiological techniques in order to identify spinothalamic tract dysfunction (e.g., cortical contact heat evoked potentials (CHEPs), laser evoked potentials (LEPs), quantitative sensory testing (QST)) might offer new insights, particularly in the field of neuropathic pain. These methods are under investigation and might proof more sensitive compared to the ISNCSCI “impaired” dermatome classification [33, 63–66, 74, 79, 97, 98, 100, 101, 146, 165]. One problem with CHEPs and LEPs however is that dephasing/desynchronization of afferent impulses makes it difficult to evoke cortical responses from long pathways (e.g., from the trunk and legs).
Regarding a deeper analysis of clinical complete SCI, routine SSEP and MEP recordings are of limited sensitivity. In this context, some specialized analyses of “discompleteness” were suggested [54, 122, 153]. The technical neurophysiological basis for these analyses is a brain motor control assessment (BMCA). This technique quantitatively analyzes the central nervous system control over spinal motor output [154]. The practical applicability to clinical routine and its meaningfulness for clinical routine have yet to be determined.
References
1.
Allert ML, Jelasic F (1969) Der Analreflex im Elektromyogram der Blasen- und Darmschliessmuskeln. Wiener Zeitschrift für Nervenheilkunde und deren Grenzgebiete 27:281–287PubMed
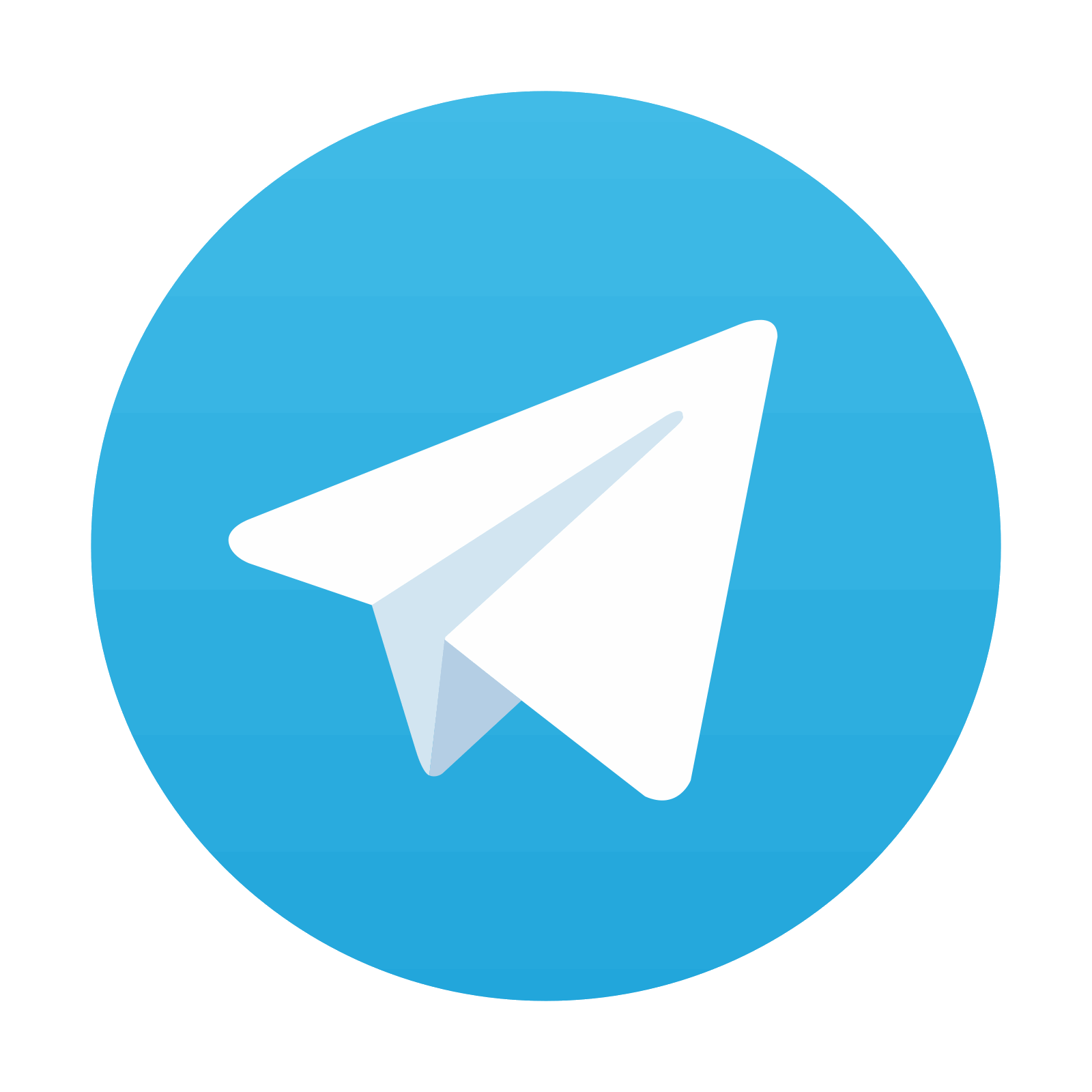
Stay updated, free articles. Join our Telegram channel
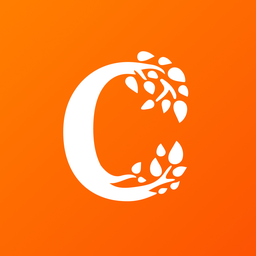
Full access? Get Clinical Tree
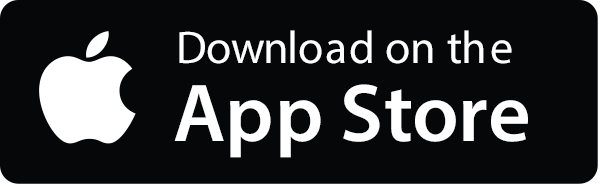
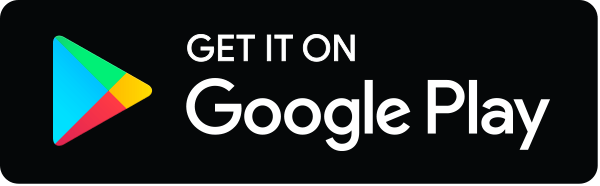