Chapter 5 Spinal Cord Stimulation
General Indications
Establishing Diagnosis
Spinal cord stimulation (SCS) is an adjustable, nondestructive, mode of neuromodulation that delivers therapeutic doses of electrical current to the spinal cord for the management of neuropathic pain. The most common indications include postlaminectomy syndrome, complex regional pain syndrome (CRPS), ischemic limb pain, and angina (Table 5-1); but it has been applied to other causes of intractable pain.
Table 5-1 Spinal Cord Stimulation Indications and Probability of Success
Indications | Probability of Success |
---|---|
Postlaminectomy syndrome/failed back surgery syndrome (FBSS) | 50%-60% with >50% pain relief |
Complex regional pain syndrome (CRPS) | 67%-84% |
Chronic critical limb ischemia (CCLI) and pain | 70%-80% with >75% pain relief |
Angina | No data available |
Abdominal/visceral pain syndromes | |
Brachial plexitis/neurogenic thoracic outlet syndrome | |
Phantom limb pain | |
Intractable pain secondary to spinal cord injury | |
Mediastinal pain | |
Cervical neuritis | |
Postherpetic neuralgia |
Anatomy
Stimulation of large myelinated afferent fibers may occur in four different areas: the dorsal root, the dorsal root entry zone (DREZ), the dorsal horn, or the dorsal columns (Fig. 5-1). Electrical stimulation of these structures results in an ipsilateral tingling paresthesia, but changes to discomfort and pain with increasing stimulation voltage. Particularly, activation of the dorsal roots causes a radicular paresthesia at a particular dermatomal level, whereas stimulation of the dorsal columns causes paresthesia in areas of the body caudal to the level of the electrode. In the cervical and thoracic cord the dorsal columns are usually about 3 to 5 mm wide, largest at the level of the cervical enlargement, and smallest in the midthoracic spine.
The single most important factor in determining stimulation parameters is the width of the cerebrospinal fluid (CSF) space dorsal to the spinal cord (Fig. 5-2). Increasing thickness of the dorsal CSF (dCSF) layer leads to early stimulation of the dorsal root fibers instead of the dorsal column fibers. This is largely because rootlet fibers, which are immersed in well-conducting CSF, have high conductivity at the DREZ. Therefore the stimulation threshold for the segmentary system ranges from 0.1 V to 0.5 V, whereas activation of the dorsal columns occurs at a threshold that is at least 0.5 V to 1 V higher.
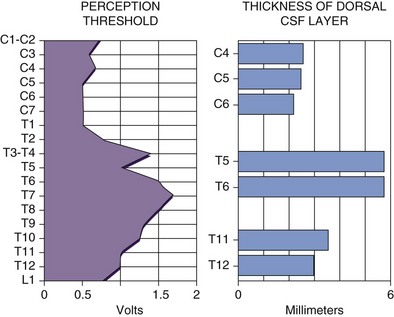
Fig. 5-2 Relationship between spinal level and perception threshold/dorsal cerebrospinal fluid thickness.
Differentiating between stimulation of the dorsal root, DREZ, or dorsal horn can be exceedingly difficult. One can expect dorsal root stimulation with laterally placed electrodes; stimulation of the DREZ or dorsal horn is more likely if the electrode is placed near midline. In the latter group stimulation leads to segmentary paresthesias that are rapidly followed by activation of the dorsal columns with a small voltage increment. Interestingly, minute changes in mediolateral position of the electrode have been shown to lead to movement of the paresthesias in a W two-dimensional pattern1 as shown in Fig. 5-3.
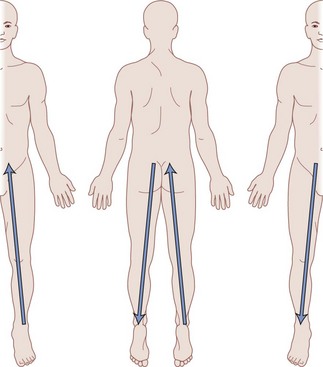
Fig. 5-3 Migration of paresthesias with mediolateral adjustment of stimulation.
Modified from Oakley JC et al: Transverse tripolar spinal cord stimulation: results of an international multicenter study, Neuromodulation 9(3):192-203, 2006.
If electrical stimulation reaches the ventral cord, motor structures are affected, and consequently muscle contractions are seen. Much like dorsal cord stimulation, activation of the ventral root or motor neurons causes muscular contractions in a myotomal distribution, whereas activation of the descending corticospinal pathways results in diffuse muscle contractions caudal to the level of the electrode. In the vast majority of patients stimulation over the dorsal columns is not complicated by simultaneous activation of the motor system unless a significantly higher voltage is implemented. Nevertheless, there have been cases in which activation of the motor structures has been seen to occur at the same threshold as the sensory system; therefore selective stimulation of the dorsal column could not be successfully maintained.2
Understanding the somatotopy of the spinal cord is paramount to knowing the technical aspects of implantation. A basic tenet of SCS is to create an area of paresthesia that overlaps the patient’s region of pain.3 Therefore correlation of the somatotopy and the level of the spinal cord is necessary. Barolat and colleagues4 have published extensively on the mapping of the spinal structures, which led to a database correlating areas of sensory response to levels dorsal SCS.4
Recently this paradigm has been challenged by case series demonstrating successful four-limb paresthesia in patients receiving only cervical stimulation.5 In general, this effect was achieved with stimulation with longer pulse widths and lower frequencies. Although the mechanism is not yet well understood, some have hypothesized that thinner dorsal CSF thickness in the cervical spine may allow for the stimulation of deeper medial and midline fibers that correspond to the lower extremities.
Basic Science
The enthusiasm with SCS began with the introduction of the gate control theory for pain control by Melzack and Wall6 in 1965. They noted that stimulation of large myelinated fibers of peripheral nerves resulted in paresthesia and blocked the activity in small nociceptive projections. In other words, appropriate stimulation of a competing afferent signal can effectively block an existing pain signal.
Although a large body of work has been published, the exact mechanisms of action of SCS remain unclear. The computer modeling work of Coburn,7,8 Coburn and Sin,9 Holsheimer and associates,10 Holsheimer and Strujik,11 and Holsheimer and Wesselink12 have shed some light, at least theoretically, on the distribution of the electrical fields within the spinal structures. It is clear that stimulation on the dorsal aspect of the epidural space creates complex electrical fields that affect a large number of structures, including afferents within the peripheral nerve, dorsal columns, or supralemniscal pathways. Mechanisms at distinct levels of the central nervous system apart from the spinal cord are believed to contribute to the effects of SCS. Such mechanisms include antidromic action potentials that pass caudally in the dorsal columns to activate spinal segmental mechanisms in the dorsal horns and action potentials ascending in the dorsal columns activating cells in the brainstem, which in turn might drive descending inhibition. Recent work by Schlaier and associates13 demonstrated changes in cortical excitability with SCS, which is believed to be N-methyl-d-aspartate (NMDA)-related neuroplasticity at the supraspinal level. At the chemical level, animal studies suggest that SCS triggers the release of serotonin, substance P, and γ-aminobutyric acid (GABA) within the dorsal horn.14–16
Indications/Contraindications
Another important consideration is the need for careful psychological screening in the selection of candidates for SCS procedure.17–24 This becomes particularly important because mood disorders can alter pain reporting and perception by a patient. Patients who have frank psychiatric disorders, excessive depression, anger, or unrealistic expectations may be inappropriate for SCS; however, some of these patients may become favorable candidates for SCS after appropriate psychiatric therapy. The most common indications for SCS follow.
Chronic Critical Limb Ischemia and Pain
In 1973 Cook and Weinstein25 were the first to suggest that the indications for SCS might extend beyond intractable pain control. They observed a group of patients with multiple sclerosis who underwent SCS to treat their chronic pain. Unexpectedly the patients experienced not only pain relief but also an improvement in mobility and sensory and bladder function. They incidentally found an apparent improvement in lower limb blood flow and subsequently used SCS in patients whose primary problem was peripheral vascular disease (PVD).26 These patients were found to have relief of rest pain, increased skin temperature, improved plethysmographic blood flow, and healing of small cutaneous ulcers.
Similarly, it is believed that SCS benefits patients with pain secondary to Raynaud phenomenon27 and diabetic neuropathy28 as well.
Angina
The role of SCS in the management of refractory angina pectoris seems to be very promising. There are well-documented reports in the literature revealing uniformly good results in the relief of angina pain.29–33 Further, the results have been maintained in long-term follow-up and have been substantiated by a reduction in the intake of nitrates as well. Interestingly, other findings have supported the evidence that SCS has effects that go beyond pain relief. The observations that there is less ST segment depression and that the exercise capacity, the time to angina, and the recovery time all improve with stimulation may suggest that there is a reduction in ischemia. In a positron emission tomography study, a redistribution of myocardial flow in favor of ischemic parts of the myocardium has been demonstrated as a long-term effect of SCS, both at rest and after pharmacological stress induction.34
Moreover, since the relation between pain and myocardial ischemia has not been fully clarified, we do not know whether the pain relief is caused by direct depression of nociceptive signals in the spinal cord or whether there is secondary gain from a reduction in the ischemia.35,36 On the one hand, a significant amount of work by Foreman14 has shown that dorsal column stimulation inhibits the activity of spinothalamic tract cells, which are typically activated by cardiac sympathetic afferents or intracardiac bradykinin. On the other hand, the effects of stimulation might be equivalent to those of a sympathectomy in that it inhibits a hyperactive sympathetic system. This latter mechanism has been shown experimentally in the rat by Linderoth and associates.37
Other Indications
Early studies in the 1980s demonstrated benefits of SCS for urinary incontinence, with consequent increases in continence and substantial reduction in residual urine volumes.38–42 These effects were found with stimulation of ventral sacral roots, a method that has also been shown to improve bowel function in patients with spinal cord injury.43 Trials in the 1970s reported initial benefits of this modality for phantom limb pain, but few of these patients experienced lasting results with long-term follow-up.44 Scattered reports also exist demonstrating the benefit of SCS in the treatment of intractable pain secondary to spinal cord injury pain, mediastinal pain, cervical neuritis, and postherpetic neuralgia.
Equipment
Electrical stimulation consists of rectangular pulses delivered to the epidural space through an implanted electrode via a power source. Electrodes were initially all unipolar in nature, but subsequently bipolar and tripolar arrays were developed. The initial radiofrequency (RF)-driven passive receivers have given way to implantable pulse generators (IPGs), which were first introduced in the mid-1970s. In 1980 the first percutaneous quadripolar electrode was produced; it could be reprogrammed noninvasively through an external transmitter.45 Subsequently IPGs that can be both transcutaneously charged and programmed have been developed. This most recent advance is now leading a renewed interest in the possibility of using special electrode arrays in the delivery of electrical stimulation to the spinal cord.
Percutaneous Electrodes
Electrode choice generally depends on how many segments of the spinal cord are to be covered. Although electrodes with larger spacing allow for broader coverage, closer spacing permits better steering and electric field shaping. The general trend is to use one or two quadripolar electrodes for limb pain and one or two octipolar electrodes for axial pain. Even insertion of three electrodes is being explored for better steering of current.46
Recently ANS (A St. Jude company, Plano, Tex) has introduced a slim-line plate type electrode that can be inserted percutaneously. The broader electrode base provides a surface in which fibrosis should lessen the risk of caudal electrode migration. The slimmer profile of the electrode might also have advantages in the cervical spine where spinal cord compression might be an issue. Finally, the design emulates that of a miniplate lead since the contacts are on one side with the other side being insulated, leading to a more energy efficient system (Fig. 5-4).
Paddle Electrodes
Paddle or laminotomy electrodes are offered in many sizes, shapes, lengths, spacing, and configurations of electrodes. They are primarily offered as single- and dual-column configurations; with three (tripole)- and five-column models being introduced more recently (Figs. 5-5 and 5-6). With regard to their shape, these electrodes may come with curved or hinged leads to help facilitate insertion.
The main advantage of these electrodes resides in their more inherent stability in the dorsal epidural space and lesser propensity to migrate. Some preliminary data by North and associates47 also suggest a broader stimulation pattern and lower stimulation requirements. As alluded to previously, paddle electrodes are also energy efficient in delivering electrical stimulation.
There is some theoretical evidence that shaping of the electrical field is possible with more complex electrode arrays. Current available tripole electrodes and their dimensions are shown in Table 5-2. Holsheimer and colleagues48 concluded that the transverse tripolar system enabled finer control of paresthesia because of its ability to more accurately shape the stimulating electromagnetic field. Specifically the transverse tripolar configurations allow for stimulation of the dorsal columns at higher amplitudes before the dorsal roots begin experiencing clinically significant stimulation. These electrodes are composed of a central cathode flanked by lateral anodes, which modulate the field medially or laterally, depending on their programmed voltage. In other words, the current delivered to the spinal cord can be steered not by moving the electrode but instead by simply modifying the voltage ratio between the central cathode and two flanking anodes.1 The lateral anodes create a shielding effect such that the dorsal roots are protected from stimulation; deeper activation of the dorsal columns is achieved with higher voltages (Fig. 5-7). This feature ultimately results in a wider therapeutic range, wider paresthesia coverage, and a greater probability to fully cover the painful area with paresthesia. Possible programming configurations of these tripole electrodes are shown in Fig. 5-8.
MDT Specify 5-6-5 ![]() | ANS Lamitrode Tripole 16C ![]() | |
---|---|---|
Electrode length (mm) | 4.0 | Mid: 4.1 Out: 5.8 |
Electrode width (mm) | 1.5 | Mid: 2.6 Out: 1.8 |
Longitudinal ctr. to ctr. (mm) | 9.0 | Mid: 7.0 Out: 7.0 |
Lateral ctr. to ctr. (mm) | 3.0 | 3.2 |
Electrode area (mm2) | 6.0 | Mid: 10.7 Out: 10.4 |
Rechargeable Implantable Pulse Generators
Rechargeable systems have now become available. Medtronic’s device, known as the Restore Rechargeable Neurostimulation System, uses a battery with an estimated 9-year total life span and takes about 6 hours to fully recharge. Advanced Neuromodulation Systems’ Eon device has a battery life that is currently estimated to be 7 years. Boston Scientific’s Precision device has a battery life estimated to be 5 years. A detailed comparison of the features of these rechargeable batteries is shown in Table 5-3.
In an analysis of cost between rechargeable and nonrechargeable systems, Hornberger and associates49 found that the former would require 2.6 to 4.2 fewer replacement procedures, resulting in a total lifetime savings ranging from $104,000 to $168,833. With regard to overall efficacy, a 12-month study looking specifically at the Restore rechargeable IPG demonstrated that the system was easy to use and that all patients were able to recharge it successfully. Moreover, both patient and physician satisfaction was high, with significant improvements in pain, quality of life, and functional status.50
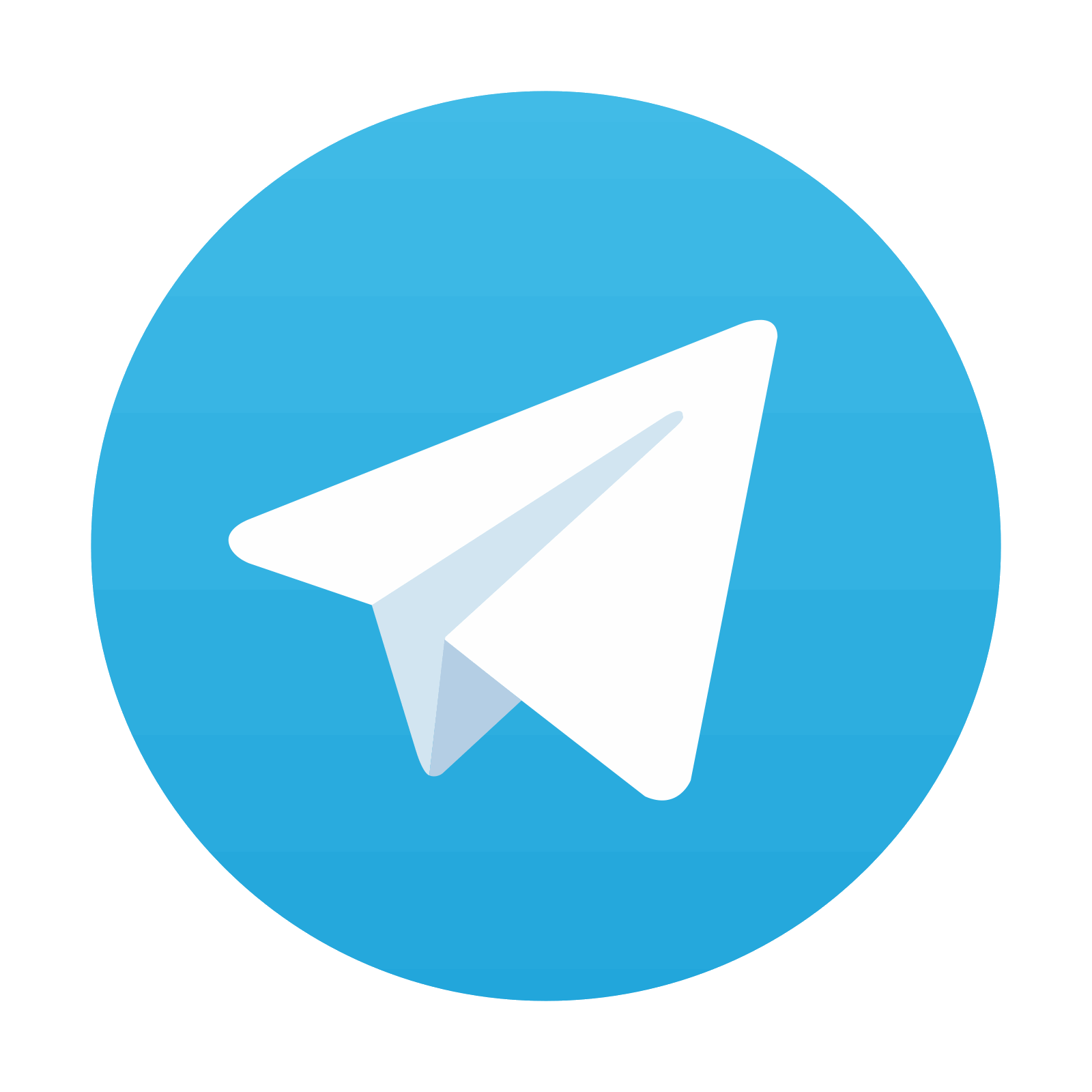
Stay updated, free articles. Join our Telegram channel
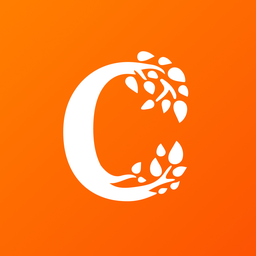
Full access? Get Clinical Tree
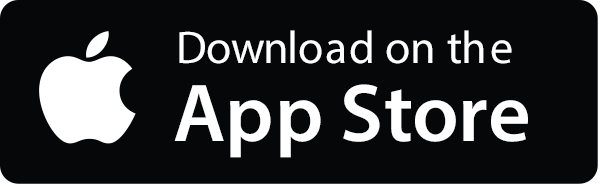
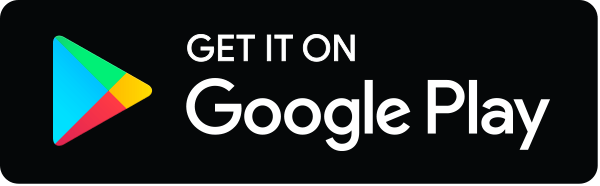