Fig. 5.1
SD and IEE. (a) SD is observed as a large negative DC shift (first trace, full-band recording between 0 and 45 Hz). (A) shows the amplitude and (B) the duration of the negative DC shift. SD is associated with spreading depression of spontaneous activity (second trace, frequency range from 0.5 to 45 Hz; third trace, power of the spontaneous activity; fourth trace, integral of the power of the spontaneous activity). (C) gives the mean amplitude of the spontaneous activity before and (D) during SD. Note the amplitude reduction (= silencing = depression) during SD. Analogously, (E)–(H) display the amplitudes of the power and integral of the power of spontaneous activity before and during SD. Note the decreases. Depression durations of SD were scored beginning at the initial decrease in the integral of the power of the AC-ECoG and ending at the start of the recovery phase (I). (b) The IEE is analyzed accordingly (J–P). Note that the IEE shows a smaller DC shift than the SD, and the amplitudes of spontaneous activity, power, and integral of the power increase during IEE in contrast to SD
The other important pathological network event in the brain is epileptiform activity (Fig. 5.1b). The so-called paroxysmal depolarization shift (PDS) characterizes interictal or preictal epileptiform activity on the cellular level. This is a synchronous event resulting from a giant excitatory postsynaptic potential that usually lasts for 80–200 ms [11]. The giant excitatory postsynaptic potential seems to be the consequence of a synchronous activation of recurrent excitatory paths. In ECoG and scalp electroencephalography (EEG) traces, its extracellular correlate is the interictal or preictal spike [12]. Ictal epileptiform events (IEEs) result from longer cellular depolarizations, which seem to be the consequence of melting PDS [13]. Clinical correlates of IEE are convulsive and nonconvulsive epileptic seizures.
From a thermodynamic perspective, IEE and SD represent two different levels of free energy between the physiological state of neurons and dead tissue. This concept has been based on the free energy contained in the electrochemical gradients across the neuronal membranes [10]. These gradients reach robust, characteristic ceiling levels during IEE and SD [14–18]. Table 5.1 shows the typical stepwise changes of electrical, sodium, calcium, and potassium transmembrane gradients in animals from the physiological state to IEE to SD. According to these changes, IEE is closer to the physiological state, while SD is closer to dead tissue. In fact, SD is the largest known disruption of viable cerebral gray matter. Nonetheless, cortical tissue spontaneously recovers from SD except under conditions of severe pathology or energy deprivation when long-lasting depolarization can lead to cell death or infarction.
Variable | Physiology | IEE | SD |
---|---|---|---|
Sustained negative neuronal membrane potential (mV) | 60–70 | 40–45 | 1–17 |
Negative intracortical extracellular DC shift (mV) | – | 1–4 | 5–30 |
Size of extracellular space (%) | 18–22 | 14 | 5–9 |
Intra-/extracellular sodium concentration (mM) | 10/146–154 | 16/135–143 | 35/57–59 |
Intra-/extracellular calcium concentration (μM) | 0.06/1200–1300 | 0.13/800–1000 | 25/80 |
Intra-/extracellular potassium concentration (mM) | 134/2.3–3.1 | 126/10–12 | 106/35–60 |
Free energy content in the electrochemical gradients across the cellular membranes of neurons (J/l) (10) | 21.9–24.4 | 19.1–21.6 | 2.4–2.5 |
Among the many variables reflecting this thermodynamic hierarchy , the negative DC shift is the one most readily measurable in humans. Here, we performed a direct comparison between DC amplitudes of IEE and SD in the human brain for the first time. The changes were similar to those previously observed in other mammals.
Methods
General
We describe four consecutive patients with aneurysmal subarachnoid hemorrhage (aSAH) who showed both IEE and SD on subdural ECoG monitoring during intensive care. The patients were prospectively enrolled at two participating centers of the Co-Operative Study on Brain Injury Depolarizations (COSBID), Campus Benjamin Franklin, and Campus Virchow-Klinikum (Charité University Medicine Berlin, Berlin, Germany). Inclusion criteria were (1) age ≥ 18 years, (2) World Federation of Neurosurgical Societies (WFNS) grades I–V , (3) ruptured saccular aneurysm proven by computed tomography (CT) angiography (CTA) or digital subtraction angiography , and (4) either surgical treatment of the aneurysm via craniotomy or, in coiled patients, burr hole trepanation for placement of a ventricular drain or oxygen sensor, which allows the simultaneous placement of a subdural electrode strip [19–21]. Exclusion criteria were subarachnoid hemorrhage due to other causes (e.g., trauma, fusiform, or mycotic aneurysm), admission in a clinical state with unfavorable prognosis (e.g., wide, nonreactive pupils for more than 1 h), bleeding diathesis or pregnancy, unavailability of the monitoring equipment, and refusal of the patient or legal representative to participate in the study. The research protocol was approved by the local ethics committee of the Charité University Medicine Berlin. Either informed consent or surrogate informed consent was obtained for all patients. Research was conducted in accordance with the Declaration of Helsinki.
Aneurysmal SAH was diagnosed by assessment of CT scans. Hemorrhage was graded according to the original Fisher scale [22, 23], and clinical presentation on admission according to the WFNS scale. A study neurologist or neurosurgeon performed a neurological and general medical evaluation on admission. Baseline demographic data and clinical signs and symptoms of the initial hemorrhage were recorded. The aneurysm was assessed using four-vessel digital subtraction angiography or a more restricted study when indicated.
After treatment of the aneurysm, all patients were transferred to the neurocritical care unit where the continuous neuromonitoring data were acquired for up to 15 days. Glasgow Coma Score, blood gases, glucose, and electrolytes were documented at least every 6 h. A thorough neurological examination was performed at least daily. Oral nimodipine was given prophylactically. Transcranial Doppler sonography was performed daily as described previously [24]. Delayed ischemic neurological deficit (DIND) was defined as the occurrence of focal neurological impairment (such as hemiparesis, aphasia, apraxia, hemianopia, or neglect) or a decrease of at least two points on the Glasgow Coma Scale (either on the total score or on one of its individual components (eyes, motor on either side, verbal) >72 h after aSAH onset). Moreover, the diagnosis of a DIND required that the neurological deficit not to have been present immediately after aneurysm occlusion lasted for at least 1 h and could not have been attributed to other causes such as hydrocephalus or rebleeding by means of clinical assessment or imaging studies of the brain and appropriate laboratory investigations [24–26]. Whenever necessary, serial CT scans were performed at times of clinical deterioration. Patients were diagnosed to have delayed cerebral ischemia (DCI) when they showed a DIND and/or a neuroimaging-proven delayed ischemic stroke [26]. Patients with DCI were treated with hyperdynamic therapy (hypertension, hypervolemia) [27]. Angioplasty or intra-arterial therapy was not performed. At the conclusion of the monitoring period, the electrode strip was removed at the bedside by gentle traction.
Recording of the Neuromonitoring Data
For the continuous ECoG recordings , a linear, six-contact platinum electrode strip (Wyler, 5 mm diameter; Ad-Tech Medical, Racine, Wisconsin, USA) was placed on cerebral cortex accessible through craniotomy during aneurysm surgery as described previously [20]. Ground was provided by a subdermal platinum needle ipsilateral to the recording strip (Technomed Europe, Maastricht, Netherlands). Full-band ECoG, including both DC and alternating current (AC) components , was recorded in six active channels from the six-contact linear electrode array (interelectrode distance 1 cm) with a subdermal platinum needle serving as reference. Monopolar recordings were acquired with a BrainAmp amplifier (Brain Products GmbH, Munich, Germany) as reported previously [20, 28]. Data were sampled at 200 Hz and recorded, filtered (0–45 Hz), and analyzed with a PowerLab 16/SP analog/digital converter and Chart-7 software (ADInstruments, New South Wales, Australia). Intracranial pressure (ICP) was monitored via ventricular drainage catheter or ICP transducer (Codman or Camino systems). The systemic arterial pressure was continuously recorded via a catheter in the radial artery. Mean arterial pressure (MAP) and cerebral perfusion pressure (CPP) were calculated according to standard practice.
Analysis of Neuromonitoring Data
In accordance with the analytical framework and characteristics described previously [24, 29, 30], SD was observed in the DC-ECoG (bandpass, 0–0.05 Hz) as the consecutive onset of a large negative DC shift in neighboring ECoG channels.
In contrast to DC changes that define the phenomenon of SD, spontaneous and evoked electrical activity of the brain is observed in the AC range of the ECoG above ~0.5 Hz. In electrically active tissue, SD typically causes spreading depression of spontaneous activity. This is observed in neighboring ECoG channels as a rapidly developing reduction in amplitude, power, and integral of the power of the AC-ECoG activity (bandpass, 0.5–45 Hz) as shown in Fig. 5.1a [31].
Depression durations of SD were scored beginning at the initial decrease in the integral (60 s decay time constant) of the power of the AC-ECoG and ending at the start of the recovery phase as described previously and shown in Fig. 5.1a [31].
IEEs were defined as any spikes, sharp waves, or sharp-and-slow-wave complexes lasting for 10 s or more at either a frequency of at least 3/s or a frequency of at least 1/s with clear evolution in frequency, morphology, or recording sites of the electrode strip. IEE are typically associated with an increase in the AC-ECoG power and integral of the power [24, 32, 33] and were analyzed as shown in Fig. 5.1b.
Statistics
First, the median of each variable was calculated within a given patient. Based on this, median, first, and third quartile of each variable was then calculated for the four patients. All SDs or, respectively, all IEEs within one patient were in other words treated as dependent. P ≤ 0.05 was accepted as statistically significant. Further details of the statistical analysis are given in the “Results” section.
Results
Case Reports
All patients had both aSAH and a large intracerebral hematoma. The clinical variables are summarized in Table 5.2.
Table 5.2
Cases and electrophysiological variables
Patient 1 | Patient 2 | Patient 3 | Patient 4 | |
---|---|---|---|---|
Age | 48 | 71 | 42 | 78 |
Sex | F | M | F | F |
History of epilepsy | No | No | No | No |
Location of aneurysm | MCA | ACoA | MCA | ACoA |
WFNS grade | 1 | 5 | 4 | 4 |
Fisher grade | 3 | 4 | 3 | 3 |
ICH (largest ∅, cm) | 5 | 6 | 3.5 | 5 |
Surgical intervention | Clip ligation | Clip ligation | Clip ligation | Clip ligation |
Total recording time of monopolar DC/AC recordings (h) | 138.6 | 226.8 | 195.6 | 210.8 |
Number of SDs in the monopolar DC/AC recordings | 58 | 114 | 28 | 15 |
Median amplitude of the negative DC shift of SD (mV) (n = local measurements) | 6.3 (4.8, 7.6) (n = 158) | 5.7 (3.9, 8.0) (n = 261) | 11.9 (8.2, 3.5) (n = 90) | 10.5 (7.6, 12.7) (n = 30) |
Median duration of the negative DC shift of SD, minimum and maximum duration (s) | 146 (116, 179) 52–418 | 159 (129, 111) 59–359 | 198 (157, 215) 108–361 | 104 (93, 123) 67–154 |
Median monopolar depression duration in the integral of power (s) | 505 (345, 675) | 501 (251, 909) | 505 (332, 720) | 397 (321, 611) |
Depression of AC-ECoG power during SD in percent of baseline (=100%) | 11 (6, 22) | 70 (35, 87) | 18 (10, 29) | 52 (31, 81) |
Depression of the AC-ECoG integral of power during SD in percent of baseline (=100%) | 11 (4, 20) | 58 (37, 75) | 18 (9, 31) | 33 (24, 37) |
Recording sites involved in SD | Up to 4 | Up to 3 | Up to 4 | Up to 3 |
Median of mean MAP during SD (mmHg) | 98 (93, 105) | 97 (92, 101) | 95 (88, 104) | 81 (78, 86) |
Median of mean ICP during SD (mmHg) | 1 (1, 1) | 15 (11, 18) | 12 (8, 16) | 11 (8, 15) |
Median of mean CPP during SD (mmHg) | 98 (92, 104) | 82 (76, 87) | 80 (75, 93) | 71 (65, 74) |
Number of IEEs in the monopolar DC/AC recordings | 15 | 34 | 127 | 195 |
Median amplitude of the negative DC shift of IEE (mV) (n = local measurements) | 0.2 (0.0, 1.0) (n = 30) | 1.0 (0.5, 1.6) (n = 71) | 0.9 (0.6, 1.2) (n = 219) | 0.0 (0.0, 0.3) (n = 319) |
Median duration of IEE (s) | 201 (94, 278) | 367 (241, 434) | 55 (34, 82) | 114 (77, 153) |
Increase of AC-ECoG power during IEE in percent of baseline (=100%) | 204 (166, 272) | 1659 (727, 2865) | 254 (169, 359) | 536 (377, 834) |
Increase of the AC-ECoG integral of power during IEE in percent of baseline (=100%) | 203 (169, 231) | 1418 (627, 2197) | 165 (140, 223) | 433 (292, 688) |
Recording sites involved in IEE | Up to 2 | Up to 4 | Up to 3 | Up to 2 |
Median of mean MAP during SD (mmHg) | 100 (98, 104) | 99 (94, 104) | 92 (89, 96) | 80 (78, 86) |
Median of mean ICP during SD (mmHg) | 1 (0, 1) | 15 (12, 19) | 17 (14, 19) | 13 (9, 15) |
Median of mean CPP during SD (mmHg) | 100 (97, 103) | 84 (76, 88) | 75 (72, 79) | 68 (64, 74) |
Patient 1 showed a DIND characterized by altered consciousness, diplopia, left facial palsy, and left arm paresis. She was treated with hyperdynamic therapy. In addition, lorazepam was given during this time period because she reported anxiety and agitation. No clinical seizures were observed. She slowly improved after day 10 without evidence of a delayed infarct on follow-up neuroimaging.
Patient 2 showed neither DIND nor delayed infarct. However, early in the course, he developed hydrocephalus, which was initially treated with extraventricular drainage. Later on, a ventriculoperitoneal shunt was established. Chest x-ray was significant for pneumonia on day 4. Pneumonia and a urinary tract infection were treated with antibiotics. On day 12, the nurses noted spells associated with breath-holding that were interpreted as epileptic seizures, since the ECoG showed IEE. The patient was treated with levetiracetam. After discharge, he developed posthemorrhagic epilepsy.
Patient 3 had a history of migraine with olfactory auras for over 20 years. During the monitoring phase, she had two typical migraine attacks, but developed neither DIND nor delayed infarct. There was no clinical evidence of epileptic seizures. She did not receive any anticonvulsant medication.
Patient 4 had neither DIND nor delayed infarct nor clinical seizures. Pulmonary function was severely compromised, and the patient progressively developed a severe hepatorenal syndrome. She died on day 12.
Comparison Between SD and IEE
A total of 215 SDs and 371 IEEs were observed and analyzed during a total ECoG recording time of 771.8 h in these four patients. Figures 5.2–5.5 display the typical electrophysiological signatures and Fig. 5.6 the time courses of SD and IEE for each patient including the time points of treatment with anticonvulsant drugs. The electrophysiological variables are summarized in Table 5.2.






Fig. 5.2
Example traces of SD and IEE in patient 1. IEE with 3/s spike-wave activity followed by SD. The star in the third trace marks the time period which is shown in the lowest trace at higher temporal resolution

Fig. 5.3
Example traces of SD and IEE in patient 2. IEE with rhythmic 2.5/s delta activity followed by SD. The star in the second trace marks the time period which is shown in the lowest trace at higher temporal resolution

Fig. 5.4
Example traces of SD and IEE in patient 3. IEE with rhythmic 3/s spikes followed by SD. The star in the fourth trace marks the time period which is shown in the lowest trace at higher temporal resolution

Fig. 5.5
Example traces of SD and IEE in patient 4. IEE with rhythmic 1.7/s spikes followed by SD. The star in the second trace marks the time period which is shown in the lowest trace at higher temporal resolution

Fig. 5.6
Occurrences of SD and IEE over time in patients 1–4. Note the predominance of SD over IEE in all patients
The median amplitude of the negative DC shift of SD was 14 times larger than that of IEE (8.4 (6.2, 10.9) mV (first quartile, third quartile) versus 0.6 (0.2, 0.9) mV, P = 0.029, n = 4 patients, Mann-Whitney Rank Sum Test). The median duration of the negative DC shift of SD was 153 (136, 169) s (n = 4 patients), and the median monopolar depression duration of SD was 503 (475, 505) s (n = 4 patients). The depression duration always exceeded the duration of the negative DC shift . During spreading depressions, the power fell from 100 to 35 (16, 57) % and the integral of power from 100 to 26 (16, 39) % (n = 4 patients). The median duration of IEE was 158 (99, 243) s (n = 4 patients). During IEE, the power increased from 100 to 395 (242, 817) % and the integral of power from 100 to 318 (194, 679) % (n = 4 patients). Overall, the analysis of percent changes in the integral of power was less robust (more artifact laden) than the analysis of percent changes in the power (SD, 370 versus 494 successful measurements at different electrodes; IEE , 624 versus 634). In the pooled analysis of all SD and IEE, linear regression found that the changes in power and integral of power significantly correlated with each other during both SD and IEE (SD, adjusted R 2 = 0.613, P < 0.001, n = 349 local measurements; IEE, adjusted R 2 = 0.470, P < 0.001, n = 622 local measurements). No significant differences in mean MAP, mean ICP, or mean CPP were found between SD and IEE (n = 4 patients) (Table 5.2).
Discussion
Comparison of IEE and SD
On the surface of the human brain, we observed that the median amplitude of the negative DC shift was 14 times larger during SD than during IEE. This corresponds well to recordings in animals in vivo and in brain slices [2, 34–36]. Full-band recordings using platinum electrodes were already performed previously to measure the large DC shift of SD on the surface of the human brain [20, 28–30, 37], and the results presented here were similar. However, such recordings have not yet been performed in patients during IEE to our knowledge. Gross and colleagues used an amplifier with a high-pass filter of 0.01 Hz and stainless steel subdural electrodes [38], which are characterized by worse low-frequency recording properties than platinum electrodes [39]. These authors did not observe low-frequency shifts with most IEEs. Ikeda and colleagues used platinum electrodes similar to us and found low-frequency shifts with most IEEs, but the amplifier was yet equipped with a high-pass filter in contrast to the present study [40]. Such a filter distorts the DC signal and causes an artificial reduction in amplitude [41].
In general, noise and movement artifacts limited the interpretation of SD and IEE in a similar fashion in the present study. However, our results suggest that it should be easier for neurophysiologists and neurointensivists to learn the assessment of SD than the assessment of IEE, given the size and the duration of the events and the more restricted number of SD versus IEE variants. Admittedly, this may sound paradoxical in view of the previous difficulties to record SD and the resulting debates of the past whether or not SD occurs in the human brain at all [42].
The difference in DC shift amplitudes between SD and IEE reported here roughly reflects the differences in ionic concentration changes and free energy between these two types of pathological network events (Table 5.1). However, there are not only quantitative but also qualitative differences in the generation of DC shifts between IEE and SD. Accordingly, SD was associated in hippocampal slices with a very large current sink located in the layer of apical dendrites, maximal among the proximal segment of dendrites, to which the cell body layer served as a source [43]. By contrast, a sink limited to the cell body layer was observed throughout the duration of IEE . It is assumed that the DC shift of IEE reflects, in particular, longitudinal and transmembrane current loops between the glial syncytium and the extracellular space, which are largely driven by the inhomogeneous distribution of the extracellular potassium concentration [44]. Further, somatodendritic neuronal dipoles are involved due to transmitter-dependent and excitation-dependent depolarization [45]. During SD, regional depolarization in single neurons seems to be the predominant factor. Following the core conductor theory, the regional depolarization establishes longitudinal gradients of neuronal depolarization and transmembrane current loops that sum up in the extracellular space to build the negative DC shift of SD [46]. This is possible because pyramidal cells are tightly arranged in parallel, thus optimizing the extracellular addition of currents and the spatial matching of single cell to population events with subcellular accuracy.
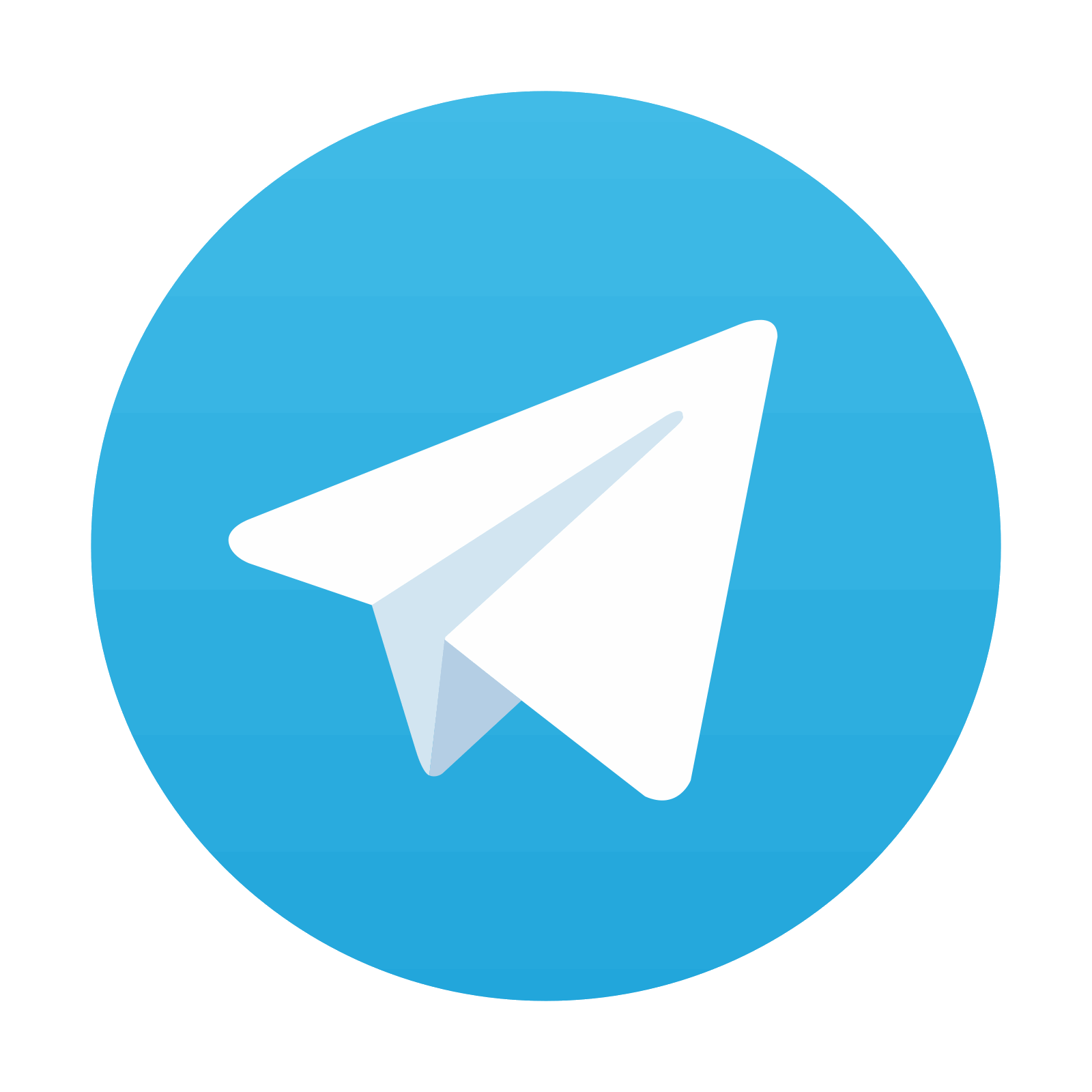
Stay updated, free articles. Join our Telegram channel
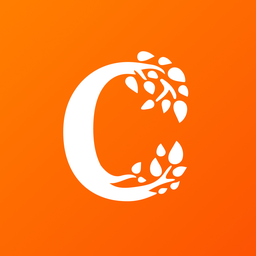
Full access? Get Clinical Tree
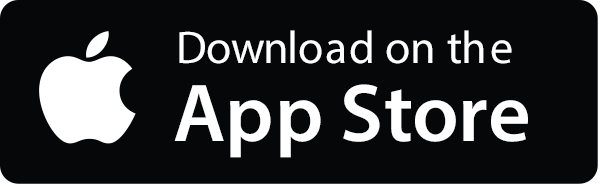
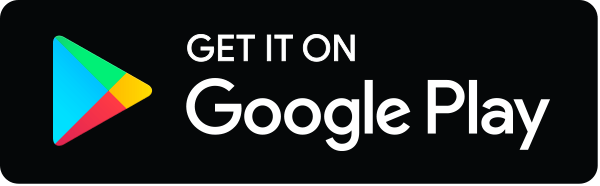