Abstract
Amyotrophic lateral sclerosis (ALS) is a challenging disease characterized by progressive loss of motor neurons. This is manifested in deterioration of strength, gait, speech, swallowing, respiratory function, and eventually leads to death. A major hurdle in development of effective therapy is rooted in the multifactorial nature of ALS pathophysiology. Cell-based therapies, in particular stem cells, have emerged as a promising therapeutic option. Stem cells have the capacity to modulate the local environment by multiple simultaneous mechanisms and promote survival of motor neurons. In this chapter, we describe various stem cell types that have been used in ALS and the underlying rationale. We also review early clinical trials using these diverse types of stem cells. Advances in stem cell therapy are moving forward at breakneck pace, and if approached with attention to methodology and regulation, have the potential to significantly improve the care of patients with ALS.
Keywords
Amyotrophic lateral sclerosis, stem cells, transplantation, spinal injection, embryonic stem cells, mesenchymal stem cells, umbilical cord stem cells, olfactory stem cells, neural progenitor cells, clinical trials
Outline
Introduction 208
Stem Cells in Amyotrophic Lateral Sclerosis: Microenvironment Modulation 209
Embryonic Stem Cells 211
Bone Marrow-Derived Mesenchymal Stem Cells 212
Peripheral Blood Stem Cells 214
Umbilical Cord Blood Stem Cells 215
Olfactory Ensheathing Cells 216
Neural Progenitor Cells 217
The Future of Stem Cells in Amyotrophic Lateral Sclerosis 222
Conclusions 223
Acknowledgments 223
References
Introduction
Amyotrophic lateral sclerosis (ALS) is a terrifying diagnosis that strikes at the core of an individual’s function and productivity. The disease is characterized by progressive and selective degeneration of upper and lower motor neurons. This manifests as an insidious, inexorable decline in motor function, with progressively compromised strength, coordination, gait, swallowing, speech, and respiratory function. For nearly all those afflicted, this means first relying on canes or walkers, then wheelchair-dependence, progressing to ventilator-dependence and a bedbound status, all the while with sensation and cognition preserved. Complications arising from this decline lead to death in an average of 3–5 years from time of diagnosis. The estimated cost of this illness to society ranges from $256–433 million to over $1 billion, yet few treatment options exist. The only US Food and Drug Administration (FDA)-approved pharmacotherapy, riluzole, extends life span by a matter of mere months. In the more than two decades since approval of riluzole, no disease-modifying therapies have come to fruition.
The reasons behind this lack of progress lie in the fact that mechanisms of motor neuron loss in ALS remain a mystery. Many models exist to explain how motor neurons are selectively killed including excitotoxicity, loss of neurotrophic factors, impaired RNA metabolism, protein aggregation, inflammatory signaling, mitochondrial pathology, endoplasmic reticulum dysfunction leading to misfolded proteins, and many others. Cases with genetic underpinnings offer some clues, with approximately 15% of cases associated with mutations in genes such as Cu 2+ -Zn 2+ superoxide dismutase-1 (SOD1), transactive response DNA-binding protein 43 (TDP43), fused in sarcoma, and the recently identified hexanucleotide repeat in chromosome 9 open reading frame 72 (c9orf72). Indeed, various animal models expressing these mutations, particularly the mutant SOD1 protein, have been used in most of the preclinical studies described. However, the large majority of ALS cases appear sporadic in nature and many treatments targeted at the above-mentioned pathways have failed in large-scale clinical trials.
In this stark landscape of ALS therapeutics, stem cells have emerged as a promising new strategy to combat the multifaceted pathology of this disease. In the short time since stem cells were first described, there has been exponential growth in scientific understanding of stem cell biology as well as potential applications in disease. In this chapter, we will begin by describing the prevailing rationale underlying stem cell therapy, then touch on current experience using different types of stem cells in ALS.
Stem Cells in Amyotrophic Lateral Sclerosis: Microenvironment Modulation
Stem cells are defined by the fundamental property of being able to undergo asymmetric cell division, with one daughter cell able to differentiate into one or more different specific cell types while the other daughter cell retains the capacity for self-renewal. There are a number of different cell types that fall under the category of “stem cell,” each with unique properties and varying degrees of differentiation potential ( Fig. 9.1 ).

Early interest in using stem cell technology in ALS was focused on the possibility of regenerating the lost motor neuron pool. Experiments using murine stem cells showed that, after in vitro exposure to retinoic acid and the hedgehog agonist Hh-Ag1.3, these cells had the ability to differentiate into motor neurons and form neuromuscular junctions after transplantation into chick embryos. However, this enthusiasm was quickly tempered, as this initial success was not reproducible in rodent models of ALS. While stem cells can indeed be induced to form motor neurons in the spinal cord, these cells must integrate into local circuitry and receive input from interneurons and descending axons, grow new axons through an impermissible central nervous system (CNS), travel significant lengths in the periphery to connect with skeletal muscle, achieve remyelination, and finally form mature neuromuscular contacts. And while these are the minimum criteria for the creation of new motor units, successful functional improvements depend on formation of sufficient numbers of motor units on proper agonist/antagonist muscles within a time window prior to irreversible atrophy of muscle tissue. With our current understanding and abilities, this goal of motor system reconstruction is currently out of reach.
Furthermore, motor neuron replacement strategies face another challenge regarding inherent ALS pathophysiology. It is now understood that while motor neuron loss is the most obvious marker of ALS, factors within the motor neuron microenvironment contribute to the pathology. In chimeric mice expressing a mutant SOD1 protein in either motor neurons or astrocytes, motor neuron survival was preserved, despite mutant SOD1 expression, if surrounding astrocytes expressed the normal gene. In contrast, motor neurons expressing the wild-type SOD1 protein still exhibited degeneration if neighboring astrocytes expressed mutant SOD1. This has led to the widely held notion that motor neuron death in ALS is not a cell autonomous process. Accumulating evidence suggests interactions with interneurons, microglia, inflammatory signaling, vasculature, Schwann cells, and skeletal muscle play a role in ALS pathology. Transplanted motor neurons would still need to survive in this hostile environment in order for cell replacement to succeed.
Given this more nuanced and global understanding of ALS pathology, stem cells still rise to the forefront as a promising therapeutic option for ALS. Stem cells can succeed where pharmacotherapy has failed by having simultaneous impact on the multifactorial pathways leading to motor neuron death. Pharmacologic therapies often achieve their effects by targeting one specific signaling pathway, and pharmacologic action in nonaffected tissues may give rise to side effects. Stem cells, on the other hand, can act locally via intercellular and paracrine mechanisms, while retaining the ability to simultaneously influence many signaling cascades that may be deranged. Thus, the majority of current stem cell therapy investigations are aimed at modulating the local microenvironment and preventing the further loss of motor neurons.
Nearly every type of stem cell has been applied in ALS animal models ( Fig. 9.1 ), including embryonic stem cells (ESCs), olfactory ensheathing cells (OECs), bone marrow-derived mesenchymal stem cells (MSCs), peripheral blood stem cells (PBSCs), umbilical cord stem cells (UBSCs), and neural progenitor cells (NPCs). While results and progress in translation to humans have been variable, the fervor with which stem cell therapy in ALS is being pursued conveys a general sense of excitement and optimism.
Embryonic Stem Cells
The cell with the most diverse differentiation capability is the ESC. This cell, derived from the embryonic blastocyst, is considered totipotent, capable of forming all tissue types if given the correct environment. While the original interest in ESCs was for motor neuron replacement, it was found that human ESCs inefficiently formed mature motor neurons. Rather, these implanted cells were locally neuroprotective, perhaps through local secretion of transforming growth factor α and brain-derived neurotrophic factors.
The robust replicative potential of ESCs is in some ways a double-edged sword. Early use of ESCs in the mutant SOD1 rat model demonstrated tumor formation at sites of cell implantation ( Fig. 9.2 ). Later explorations of ESCs in this and other models of motor neuron degeneration would utilize in vitro differentiation of murine ESCs toward motor neuron fates prior to implantation.

Part of the difficulty with using ESCs also involves the social, ethical, and political ramifications of establishing and utilizing new cell lines derived from human embryos. Therefore, while ESCs possess extraordinary potential, the attendant caveats have made ESCs a less attractive option for clinical translation. To date, no clinical trials in humans have been conducted using human ESCs.
Bone Marrow-Derived Mesenchymal Stem Cells
Another well-described pool of stem cells are MSCs that are enriched in bone marrow. These cells normally support hematopoietic stem cells in the bone marrow, contribute to formation of mesenchymal tissues (adipocytes, chondrocytes, osteocytes, etc.), and can even transdifferentiate to cell fates of nonmesenchymal origin. Despite the capacity to form new neural and glial tissue, the usefulness of MSCs likely lies in their immunomodulatory characteristics and their role as paracrine “feeder” cells. Experiments with transplantation of bone marrow MSCs in ALS models demonstrated immune modulation and microglial formation, as well as neurotrophic support by glial derived neurotrophic factor, vascular endothelial growth factor, and other growth factors. These studies have demonstrated increased motor neuron survival, as well as functional improvements by a variety of physiological measures, and have been repeated using rat or human MSCs in mutant SOD1 animal models with similar findings.
An advantage of using bone marrow-derived MSCs is the presence of well-established experience in harvesting and expanding clinical-grade MSCs from patient bone marrow. Pilot studies using infusion of MSCs have demonstrated tolerability and safety. In one study of 10 patients receiving intrathecal MSCs harvested by bone marrow aspiration, no serious adverse events (AEs) were reported, and some showed stabilization of disease by the ALS Functional Rating Score-Revised (ALSFRS-R). Another study enrolled 19 patients who received intrathecal MSCs harvested by bone marrow aspiration. No significant AEs were reported and a 6-month period of disease stability was seen after the procedure. Building on the idea of immune-mediated neurotoxicity and immunomodulation, T-cell vaccination was used in conjunction with MSCs differentiated along a neural precursor path. The T-cell vaccination was inspired by experiments in multiple sclerosis showing induction of immune tolerance, vis-à-vis the plethora of data supporting the role of neuro-inflammation in ALS. This protocol involves obtaining peripheral blood mononuclear cells by apheresis, immune-negative selecting for T cells, inactivating the T cells by radiation, then administering the T cells to patients by IV infusion every 28 days. Subsequently, seven patients completed a protocol involving intra-arterial bone marrow infusion, intravenous administration of autologous in vitro-expanded effector T cells, followed by intra-arterial administration of autologous MSC-derived Neural stem cells. No significant AEs were seen and some patients experienced a transient improvement in symptomatology. Another ongoing paradigm evaluating both intrathecal and intramuscular injection of MSCs modified to secrete neurotrophic factors is currently recruiting patients (BrainStorm Cell Therapeutics, NCT02017912).
More invasive intraspinal injection of bone marrow-derived MSCs has also been performed. Consecutive phase I studies in Italy studied 9 patients initially, followed by an additional 10 patients, for a total of 19 patients. In these studies, bone marrow obtained from the iliac crest was expanded in vitro prior to direct surgical implantation of the cells into the dorsal spinal cord. The procedure was well tolerated and led to a slowed disease course in some patients. Another group evaluated 11 patients following injection of autologous bone marrow MSCs into the spinal cord. No serious AEs were reported, nor were there any reported changes in the disease course; however, MNs near the areas of grafting showed fewer degenerative signs on histopathology. Finally, a study assessing 13 patients receiving bone marrow-derived MSCs by various routes indicated that the procedure was safe. In some cases motor improvement was also reported. The translation of MSCs to modulate motor neuron microenvironments appears promising and we await results of ongoing clinical trials with excitement.
Peripheral Blood Stem Cells
MSCs have also been isolated from adult peripheral blood, thus allowing clinicians to forego bone marrow aspiration. Typically granulocyte-colony stimulating factor (G-CSF) is administered to promote the mobilization of CD34+stem cells from the bone marrow into the peripheral blood. These can be collected by apheresis and expanded for later re-infusion. These CD34+PBSCs were initially found in cancer patients receiving chemotherapy, and it was later shown that these circulating hematopoietic progenitor cells could migrate into the CNS and provide support for diseased MNs. G-CSF itself has also been suggested to have a neuroprotective effect. Thus, a number of strategies have been implemented to collect and redistribute PBSCs to the CNS in ALS.
Based on the presumption that PBSCs can migrate into the CNS, a study using subcutaneous G-CSF given to 13 patients showed a slowing of disease progression by ALSFRS-R and compound muscle action potentials. Another study in 17 patients comparing subcutaneous G-CSF to a placebo showed elevated CD34+PBSCs but no difference in disease progression. Similarly, the STEMALS trial utilized subcutaneous G-CSF along with a 5-day course of mannitol with the hopes of increasing permeability across the blood–brain barrier in 26 patients. Again, there was an increase in circulating CD34+PBSCs, and decreases in the proinflammatory cytokines monocyte chemotactic protein-1 (MCP-1) and interleukin-17 (IL-17) were also noted, but no change in ALSFRS-R was detected.
Some groups have tried to enrich the number of circulating PBSCs first by collection of CD34+cells induced by G-CSF, followed by re-administration. One study utilized subcutaneous G-CSF in eight patients prior to PBSC isolation and infusion peripherally. While no AEs were reported, clinical and imaging measures did not seem to be significantly impacted. Finally, an aggressive strategy was trialed in six ALS patients who received total body radiation followed by peripheral infusion of G-CSF-primed PBSCs from human leukocyte antigen (HLA) matched siblings. Patients were immunosuppressed with methotrexate and tacrolimus, and graft versus host disease was seen in half of the patients. Although donor hematopoietic stem cells entered the CNS at sites of MN degeneration and engrafted as immunomodulatory cells, no clinical benefit was detected and the study was halted due to a lack of benefit and impaired quality of life.
In contrast to the above G-CSF studies, others have examined more invasive procedures for PBSC delivery in order to bypass the blood–brain barrier. An early study tested three patients using a protocol of subcutaneous G-CSF therapy and isolation of CD34+stem cells, with subsequent intrathecal administration of collected stem cells showing minimal AEs. A separate group has focused on CD133+cells mobilized by G-CSF with direct injection into cortical motor areas of the brain. In the initial study, 10 patients were enrolled and compared to 10 control patients not accepting treatment or who applied after the study period. The treatment group showed an improvement in baseline ALSFRS-R scores; however, this control group did have a higher ALSFRS-R score at baseline. An additional 67 patients were then evaluated after having undergone the same procedure. Two postoperative deaths were reported: one attributable to a postoperative myocardial infarction along with an acute subdural hematoma requiring operative evacuation, whereas the other was attributed to progression of disease. No long-term outcome data from this larger cohort have yet been reported.
Overall, studies with PBSCs appear to demonstrate clinical safety, with a suggestion of clinical efficacy in some cases. Based on preclinical models, this is an area that does demonstrate therapeutic potential; however, it is clear that these trials struggle with the technique of stem cell delivery, balancing widespread yet inefficient distribution of blood-borne PBSCs against expedient yet risky surgical methods. Hence, moving forward, this method of therapy will benefit from some agreement on G-CSF delivery strategies as well as with good clinical trial design utilizing large numbers of well-defined patient populations and standardized outcome measures.
Umbilical Cord Blood Stem Cells
MSCs are also found in umbilical cord blood. Soon after an infant’s delivery, blood from the placenta and umbilical cord is extracted and UBSCs can be isolated. Previously, UBSCs have found application in diverse conditions, reviewed elsewhere. They hold particular promise in neurodegenerative disease due to their immunomodulatory effects as well as their ability to differentiate into cells bearing neuronal and glial markers.
Initial work utilized intravenous administration of human UBSCs in murine models of ALS. This method demonstrated that human UBSCs could enter the CNS diffusely, and formed cells with astrocytic and neuronal characteristics. This paradigm did also appear to modestly delay disease onset along with improvements in neuromuscular function by electrodiagnostic measurement. More invasive intraventricular introduction of UCSBs also slowed motor decline and improved survival in the mutant SOD1 mouse. Initial trials with intraspinal injection were disappointing, but later injection of a population purified for CD34+ UCSBs showed clinical efficacy and improved survival.
An attempt at using UCBs in humans has been reported in one German patient. When this patient’s granddaughter was born 6 months after his diagnosis of ALS, CD34+UCBs from this infant were cryopreserved and banked. Later, these CD34+UCBs were thawed and the patient received 1.34 ×10 7 cells divided between six total injections at the T8 spinal level. For a period of about 24 months after UCB injection, the patient’s ALSFRS-R score plateaued (interestingly, effects were seen in lower extremity and bulbar function but not upper extremity function). The patient’s disease later began to progress, but no AEs were attributable to the surgical procedure.
Olfactory Ensheathing Cells
While many of the MSCs described above are more easily accessible, true CNS stem cells are less accessible and are produced in far fewer numbers during adulthood. While stem cell generation has been observed in the dentate gyrus and subventricular zone, the degree of stem cell proliferation and the difficulty in harvesting these cells make the use of these cells in human adults impractical at this time. The other more accessible site of stem cell generation in adults is the olfactory epithelium. Here, OECs regenerate olfactory neurons and supporting glial/sustentacular cells. OECs have been applied to models of spinal cord injury, stroke, Parkinson’s disease, and have been shown to modulate immune responses, provide a scaffold for regenerating axons, promote remyelination, and even differentiate into neuronal and glial tissues. Harnessing this capability has formed the rationale for using OECs in neurodegenerative diseases like ALS.
Initial studies isolated neurosphere-forming cells from mouse olfactory bulb. After transplantation in mutant SOD1 mouse spinal cords, formation of neurons expressing choline acetyltransferase, interneurons, and various glia was observed. Native motor neuron survival was increased, and mice receiving cell transplants demonstrated a delay in disease onset as well as prolonged lifespan. Remyelination may also have contributed to functional improvement in a study with mutant SOD1 rats. Implantation of OECs in the ventricular system also demonstrated dissemination of stem cells; however, in this case mutant SOD1 mice receiving OECs showed no improvement in motor performance (in contrast, this study showed an improvement in function in females that received ventricular implantation of MSCs).
Studies with implantation of OECs into the corona radiata of mutant SOD1 rats demonstrated downstream rescue of distant spinal cord motor neurons. On the basis of these data, a treatment paradigm has been implemented utilizing surgical implantation of fetal OECs into the bilateral corona radiata. Early results suggested reduction in disease progression by patient or caregiver self-report.
A larger study of 507 patients was then pursued, showing an increase in ALSFRS-R scores. In this larger study, no control groups or dose-responsive effects were reported. Some concerns have been raised regarding patients enrolled in these trials given the undefined trial protocols. One report on seven Dutch patients evaluated at a local institution demonstrated no change in disease progression. Another Spanish study on three patients undergoing this procedure again did not show a change in disease course. A postmortem analysis of two patients who underwent the procedure showed graft encasement with no evidence to suggest alteration of ALS neuropathology. Finally, one patient from the United States was reported to show disease progression and possible trauma with hemorrhage and vasogenic edema at the injection site. With these concerns raised in the literature, ongoing implementation of OECs in ALS should proceed with caution, close scrutiny, and ethical and robust clinical trial design.
Neural Progenitor Cells
NPCs are pluripotent stem cells, having a differentiation profile restricted to neuronal and glial cell types. Thus, they carry the potential for generation of CNS-relevant tissues without the tumorigenic potential of more primitive cells such as ESCs.
One such cell line, NSI-566RSC, has been extensively studied in preclinical experiments. An immortalized cell line derived from donated human fetal spinal cord, NSI-566RSC cells implanted into mutant SOD1 rats rescued motor neurons, correlating with improved motor function and prolonged lifespan. The concept of motor neuron rescue by local effects was reinforced in these studies. Neurotrophic signaling has been demonstrated, as well as formation of inhibitory synapses favoring motor neuron survival in the region of cell implantation but not distantly.
With this promising preclinical data in hand, development of techniques for intraspinal injection of stem cells was also pursued. Given the spatially restricted effect of NPCs on local motor neurons, any technique for spinal injection would require accurate and reproducible placement of cells in the anterior horn of spinal cord gray matter, while minimizing trauma to the exquisitely sensitive surrounding spinal cord. A specialized spinal cord injection platform was devised ( Fig. 9.3 ) and tested in the Gottingen minipig. Features aimed at maximizing safety for use in humans were incorporated into the design of the injection platform. These include a frame that is anchored to the bony spine, facilitating platform stability if the operative table requires adjustment or the patient exhibits any intra-operative movements. A traveling gondola carries a multi-axial “Z-drive” allowing precise adjustments in Cartesian as well as polar coordinate planes. The injection needle is configured with a hub that, when flush with the dorsal surface of the spinal cord, places the tip of the needle within the anterior horn (3–5 mm from dorsal surface). This needle is supported by flexible tubing that allows the needle to “float” with the cardiorespiratory pulsations of the spinal cord, and thus minimize trauma.

In the minipig, experiments showed that these animals tolerated 5–10 unilateral cervical spinal cord injections with all recovering to preoperative baseline neurologic function by postoperative day 14. Immunosuppressive regimens were also optimized in these animals to maximize cell survivability. This work paved the way for a pivotal Phase I clinical trial using NPCs in patients with ALS.
With supportive preclinical data for NSI-566RSC in the mutant SOD1 rat model and reassuring safety data with the spinal injection device in the Gottingen minipig, the US FDA gave approval for “A Phase l, Open-label, First in Human, Feasibility and Safety Study of Human Spinal Cord Derived Neural Stem Cell Transplantation for the Treatment of Amyotrophic Lateral Sclerosis” (NCT01348451). This study employed a “risk escalation” design to establish safety. First enrolled were nonambulatory ALS patients receiving five unilateral lumbar stem cell injections (100,000 cells in 10 μL per injection) or five bilateral (10 total) lumbar stem cell injections at the same cell dose. Then, the same implantation paradigm was used in ambulatory ALS patients. Next, five unilateral cervical injections (at the same dose) were made in ambulatory ALS patients. Finally, the cohort receiving five bilateral lumbar injections then underwent five unilateral cervical injections as the final “highest-risk” group. Safety and tolerability of the NPC implantation protocol were firmly established, with most AEs attributable to the immunosuppressive regimen required postoperatively and no complications related to the procedure itself.
In a number of patients, the slope of disease progression was noted to improve after surgery. Using a sliding-window analysis of ALSFRS-R scores, many patients demonstrated stability or even improvement in the ALSFRS-R, particularly in patients who received both lumbar and cervical injections ( Fig. 9.4 ). A majority of patients demonstrated improvement in grip strength testing, hand-held dynamometry, and electrical impedance myography. These improvements can be correlated to the implanted stem cells that were shown to survive over the long term. To date, seven deaths have been recorded among the patients enrolled in the Phase I trial (six due to ALS disease progression and one due to a previously undiagnosed congenital cardiac defect). Autopsy data demonstrated no tumor formation in any subject. Intriguingly, analysis of these spinal cords demonstrated live donor cells in recipient tissue ( Fig. 9.5 ) by histologic staining. In female subjects, fluorescence in situ hybridization for donor male XY chromosomes showed transplanted cells surviving amid recipient XX cells. Quantitative polymerase chain reaction also showed persistence of donor DNA to some degree in all postmortem specimens.

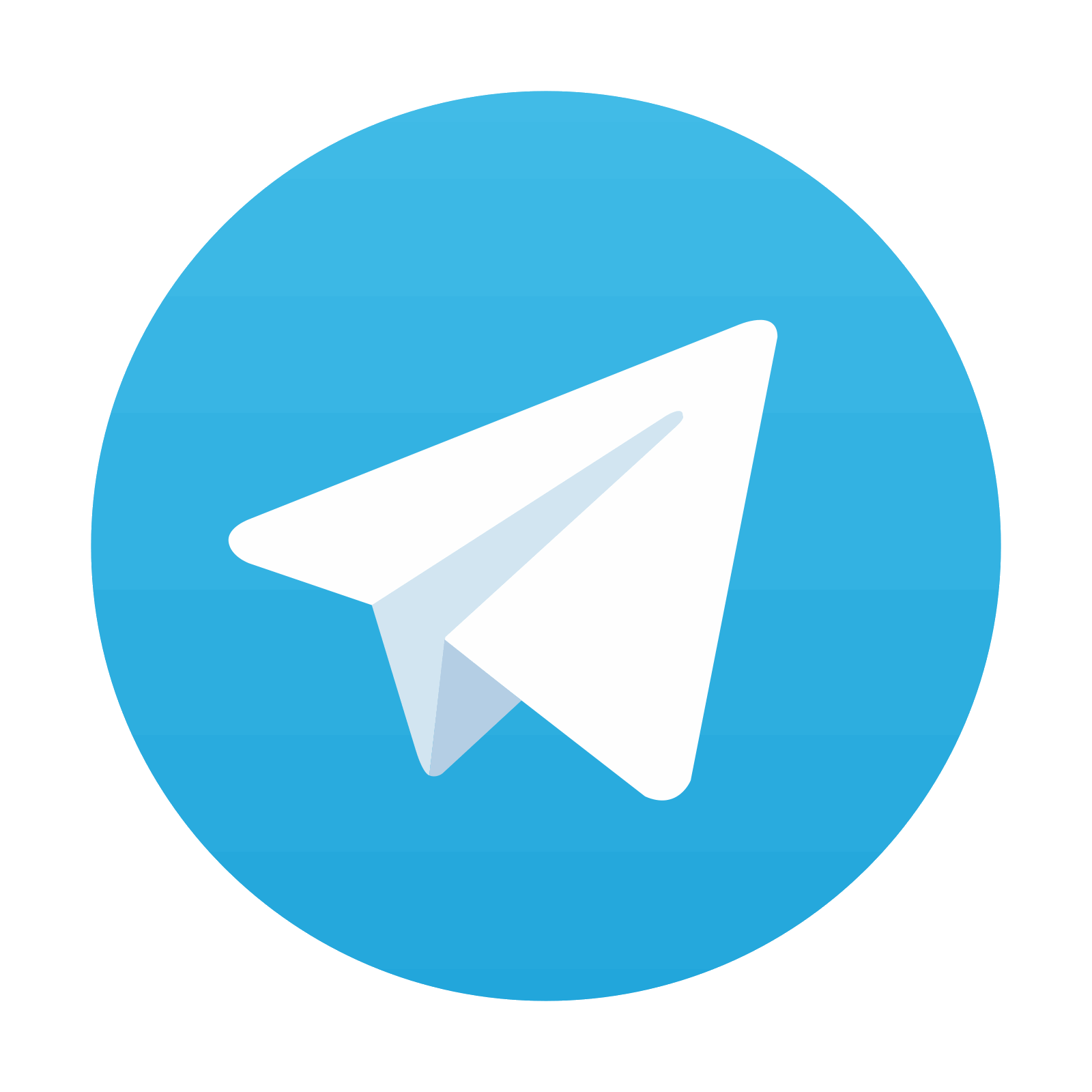
Stay updated, free articles. Join our Telegram channel
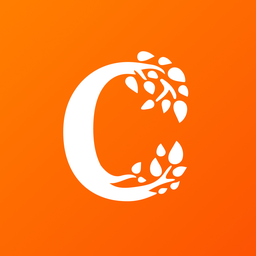
Full access? Get Clinical Tree
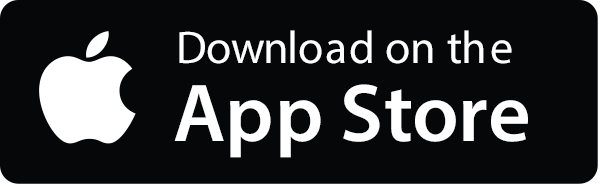
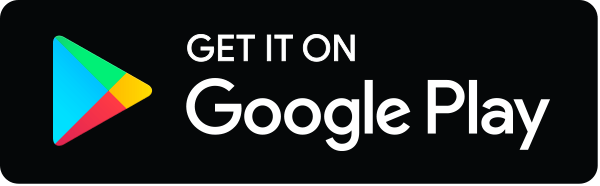
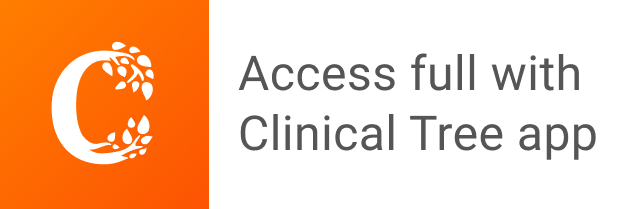