Abbreviations
ALS
amyotrophic lateral sclerosis
BDNF
brain-derived neurotrophic factor
CNS
central nervous system
DNA
deoxyribonucleic acid
EMG
electromyography
ESC
embryonic stem cell
GABA
gaba-aminobutyric acid
iPSC
induced-pluripotent stem cell
ISNCSCI
International standards for neurological classification of spinal cord injury
MSC
mesenchymal stem cell
ROS
reactive oxygen species
SCI
spinal cord injury
Acknowledgements
None.
Funding
This work was unfunded.
Disclosure statement
The authors report no conflict of interest.
Introduction
Spinal cord injury (SCI) is a devastating condition that is associated with a poor prognosis and long-lasting disability. Globally, SCI has an incidence of 8 to 246 per million and a prevalence of 236 to 1298 per million ( ). The lifetime cost for healthcare and living expenses that are attributable to SCI ranges from $1 to $4 million ( ). The destruction and demyelination of neurons that occurs in SCI leads acutely to quadriplegia, paraplegia, quadriparesis, and paraparesis, depending on the degree and location of injury. Furthermore, damage to neurons, glia, and vasculature also triggers a downstream sequence of events mediated primarily by inflammatory cells and cytokines. These events can lead to spreading of injury to adjacent segments. SCI can also lead to chronic complications, including bladder and bowel dysfunction, sexual dysfunction, neurogenic pain, and muscle spasticity. The current available treatments for SCI are limited to those that aim to ameliorate these chronic symptoms and complications ( ; ). There are currently no therapies available that produce neurological improvement for SCI patients. Thus, the development of therapies seeking to restore sensory and motor function has been an active area of investigation. One approach, stem cell transplantation, aims to replace the cells and signaling factors that are lost in SCI. In the following chapter, we will discuss stem cells, with a particular emphasis on their potential role as a therapy in SCI.
Pathophysiology of SCI
The pathophysiology underlying SCI is thought to begin with an initial insult followed by a secondary injury cascade that is characterized by three overlapping phases: acute, sub-acute, and chronic phases ( Table 1 ). The acute phase refers to the period immediately following mechanical insult that leads to compression or transection of the spinal cord. This initial event damages neurons and glial cells, disrupts membrane integrity and electrical conductance, and destroys local vasculature. These processes disturb the blood–spinal cord barrier, leading to ionic imbalance and excitotoxicity ( ). The sub-acute phase encompasses the inflammatory cascade and resultant cell death by apoptosis, ischemic necrosis, ferroptosis, and demyelination through Wallerian degeneration ( ). The chronic phase is defined by remodeling and healing which usually produces a glial scar and cystic cavity at the lesion focus ( ; ).
Stage | Key events | Consequences |
---|---|---|
Acute | Mechanical compression or transection of spinal cord |
|
Sub-acute | Triggering of inflammatory and immune responses |
|
Chronic | Remodeling and healing |
|
This influx of immune cells (such as macrophages, microglia, and neutrophils) is critical for clearing of debris and healing of the initial trauma, but they can also induce further inflammation by cytokine release and spreading of injury to adjacent segments ( ; ). In addition to perpetuating further inflammation, immune cells also generate reactive oxygen species (ROS) that can cause oxidative damage to lipids, proteins, and DNA ( ). These oxidative reactions can incite further necrosis and apoptosis ( ; ). After the inflammatory process abates, a glial scar is formed from astrocytes. This glial scar serves as a physical and chemical barrier that impedes axonal regeneration and neurite growth ( ).
Ultimately, these processes result in the loss of three components that are critical for sub-serving sensory and motor networks of the spinal cord: neurons, myelin, and neurotrophic factors. Thus, if stem cells could be directed to differentiate into neurons, oligodendrocytes that promote myelination, or neurotrophic factor-producing cells, meaningful functional and clinical recovery may be possible.
Stem cells: A brief overview
Stem cells are characterized by their capability to undergo both differentiation and self-renewal ( ). Classification of stem cells can be based on their potential differentiation into distinct cell types. Totipotent stem cells can become any cell type in the developing embryo, as well as extra-embryonic cells like the placenta. Pluripotent stem cells have a more restricted differentiation potential and are only able to make cells of the developing embryo. In other words, pluripotent cells can become any cell in the adult body. Multi-potent cells are further restricted and are only able to mature into cell types within a particular germ layer (e.g., any cell type derived from embryonic ectoderm).
Embryonic stem cells—An overview of pre-clinical findings
During development, embryonic cells can differentiate into a multitude of different cell types that compose the different organs and organ systems of an adult animal. Thus, embryonic stem cells (ESCs) were among the first reservoirs of pluripotent stem cells to be tapped for use in SCI cell-based therapy research ( Table 2 ).
Stem Cell Type | Source | Pros | Cons |
---|---|---|---|
Embryonic stem cells |
|
|
|
Mesenchymal stem cells |
|
|
|
Induced pluripotent stem cells |
|
|
|
Animal-derived ESCs
The use of rodent ESCs in animal models of SCI has produced encouraging results at the molecular and functional level. Transplanted ESCs can survive and differentiate into neurons, oligodendrocytes, and astrocytes ( ; ). Furthermore, these transplanted cells can also form new synaptic connections ( ; ). It has been shown that introduction of rodent ESCs with biomaterial scaffolding, may improve survival of transplanted cells, greater retention of cells at the locale of injury, and facilitation of formation of new circuitry ( ; ). In addition to scaffolding, survival and maintenance of stem cells may also be promoted with co-transplantation with factors, such as brain-derived neurotrophic factor (BDNF) and vascular endothelial growth factor ( ; ). Functionally, ESC transplanted cells have been demonstrated to improve locomotion in SCI models ( ; ). Lastly, there is also mounting data from SCI rodent model studies suggesting that transplanted ESCs may be able to reduce some of the long-term complications such as chronic pain and cardiovascular dysfunction ( ; ).
Human-derived ESCs
The advent of molecular protocols capable of directing human ESCs, taken from human blastocysts, to neuronal or glial fates facilitated SCI transplantation studies ( ). Demyelination is one of the hallmarks of SCI and, thus, several investigators have pursued transplanting oligodendrocyte progenitor cells to remyelinate descending spinal cord axons. In one study, Keirstead and colleagues transplanted human ESC derived-oligodendrocyte progenitors into the spinal cord of adult rats that were either 7 days or 10 months after thoracic contusion SCI ( ). Among rats that received the transplant 7 days after injury, the investigators observed differentiation of progenitors into mature oligodendrocytes, remyelination of axons, and improved motor functioning. Differentiation into oligodendrocytes was also seen in rats that were transplanted 10 months after injury. However, these rats failed to demonstrate signs of remyelination or locomotor improvement. These data indicate that the value of transplanting stem cells in SCI patients may depend somewhat on the time since injury. In another study, transplanted human ESCs were shown to further express neurotrophic factors such as BDNF and transforming growth factor-B2 ( ). These factors are, among other things, important for axonal regeneration and neurite growth and could further contribute to the utility of human ESCs as a therapeutic agent.
ESC limitations
Taken together, there is evidence suggesting that transplanted ESCs can survive, mature, reintegrate into existing networks, and promote at least modest motor restoration in vivo. ESC studies, however, have also been accompanied by several limitations. For example, in studying ESCs, they must be collected from the inner cell mass of the blastocyst. This procedure ultimately leads to the demise of the blastocyst ( ) and has raised ethical concerns ( ). Furthermore, ESC transplantation depends critically on the ability of the stem cell to differentiate specifically into a particular target cell type. When this fails to occur, one of the consequences is the generation of a teratoma, a tumor made up of somatic cells of diverse types ( ). The final limitation, as is the case for all forms of allogeneic transplantation, is that there always remains a definite concern for inciting an immunologic response against the transplanted cells ( ).
Thus, despite the encouraging findings from ESC studies that established stem cells as a promising potential SCI therapy, ESC approaches have suffered from several drawbacks that make clinical translation difficult. For these reasons, alternative sources of stem cells have been investigated.
Toward autologous transplantation: Mesenchymal stem cells
Among these alternative sources are mesenchymal stem cells (MSC), named for their ability to mature into mesoderm derived tissues, such as bone and cartilage ( Table 2 ). MSCs possess their own benefits and challenges distinct from ESCs. Because they can be derived from adult tissues, MSCs are not shrouded in the same ethical controversies as ESCs. MSCs, like other forms of stem cells, can be transplanted intravenously as they are attracted by inflammatory factors to the site of injury. Lastly, because transplantation can be autologous, MSC interventions would be less likely to elicit a significant immune response. With regards to drawbacks, one of the most critical is the limited potency relative to ESCs. Nevertheless, animal studies suggest that they can differentiate into cell types that have sufficient specificity to integrate into the spinal cord and induce functional gains.
Bone marrow–derived MSCs
Adult bone marrow harbors MSCs whose usual physiological role is replacing the cellular components of blood. The migratory ability of bone marrow–derived MSCs was demonstrated when murine bone marrow MSCs were injected into the lateral ventricles of neonatal mice and were found to migrate throughout different regions of the brain, such as the hippocampus, cerebellum, and brain stem ( ). This was followed by a study in which bone marrow MSCs were retrieved from the lower extremities of rats and centrally injected into rats up to 7 days after thoracic contusion SCI ( ). Implanted cells differentiated toward astrocytes near the site of injury and, furthermore, gait improvements were found. Notably, more favorable effects were observed when transplantation of MSCs occurred a week after, rather than immediately after injury. Finally, in a step toward assessing the utility of human adult bone marrow MSCs, another study evaluated the effect of intravenous injection of human bone marrow MSC in SCI rat models 7 days after compression injury. The authors demonstrated with immunohistochemical analysis that the intravenously introduced bone marrow MSCs migrated to the site of injury. More specifically, the MSCs integrated within the ventrolateral white matter tracts. With respect to their role within their new home, MSCs were found to differentiate into oligodendrocytes, and increased axonal growth was observed in areas adjacent to injury. Functionally, locomotor improvement was seen at weeks 3 and 4 ( ).
Adipose tissue–derived MSCs
Adipose tissue represents another tissue source with readily available MSCs. Adipose MSCs are unique in that their effect in spinal cord recovery may be mediated by changes in vasculature. One study implanted masses of adipose MSCs from humans into a rat SCI model and found that transplanted cells released angiogenic factors that ultimately led to vascular remodeling ( ). This was confirmed with another study that intravenously injected adipose MSCs from dorsal fat pads into thoracic contusion SCI model rats 8 days after injury and demonstrated angiogenesis, as well as motor recovery ( ). Another interesting feature of adipose tissue is that MSCs isolated from cryopreserved adipose tissue have been shown to exhibit similar biochemical characteristics as adipose MSCs from fresh tissue ( ). The implication here is that large stores of adipose can be collected for a tissue bank that can potentially serve as a stem cell reservoir.
Umbilical cord-derived MSCs
Lastly, MSCs derived from umbilical cord blood may also hold therapeutic potential for SCI. The utility of umbilical cell therapy for CNS injury was first introduced in the context of stroke ( ) and traumatic brain injury ( ). Given the promising results in these two contexts, their utility in SCI began to be investigated shortly thereafter. Umbilical MSCs have now been shown to promote improved motor function in thoracic compression and contusion SCI rat models ( ). Moreover, the specificity of the umbilical cord MSC’s targeting of injured cord, if introduced intravenously, has also been well demonstrated. In the study by Saporta and colleagues, umbilical MSCs were only found in the spinal cord of SCI rats at the level of injury. The introduced cells were absent from non-injured areas of the spinal cord and, moreover, were not found in the spinal cord of non-injured rats. Importantly, similar in spirit to what has been shown for bone marrow, injection of umbilical MSCs 5 days after injury yielded superior results over injection 1 day after injury ( ). It is posited that the presence of inflammatory cytokines in the hours immediately after traumatic injury restricts the engraftment of transplanted stem cells into native tissue.
Numerous animal model and basic science studies have illustrated the potential utility of MSCs. From a bench-to-bedside translation standpoint, MSCs offer significant advantages such as mobility that allows for intravenous introduction, autologous transplantation, and relatively large available stores. Although there remains some interest in MSCs for SCI therapy, a few years after the first MSC–SCI studies were reported, advances in molecular techniques ushered in an arguably more promising source for stem cells.
Cellular reprogramming: Induced pluripotent stem cells
One of the great feats of molecular biology was the discovery of factors that could be applied to adult somatic cells and cause them to dedifferentiate ( ). After application of these factors (e.g., SOX2), somatic cells are transformed into pluripotent cells. These cells, termed induced-pluripotent stem cells (iPSCs), evaded two major limitations that had hindered the progress of the development of ESC therapies. First, production of induced-pluripotent stem cells does not necessitate the destruction of a blastocyst, overcoming some of the ethical concerns tied to ESC. Second, because iPSCs can be derived from the adult tissue of an SCI patient, the subsequent transplant would be autologous and would pose lower risk for an immune-mediated transplant reaction. At the outset, iPSCs looked to be a prime candidate for SCI transplant therapy ( Table 2 ). Results from animal model studies have garnered even further advancement.
One of the first steps in attempting to design iPSC-based transplant therapies was the development of protocols that promote iPSC differentiation specifically into neurons, glia, or their precursors. Several studies have now demonstrated this capability with both murine and human iPSCs ( ; ). In addition to showing that neural and glial precursors could be produced from iPSC, there are mounting data that these cells can be integrated into motor networks after transplantation. In one study, murine iPSCs were transplanted into thoracic contusion SCI mice and differentiated into neurons, astrocytes, and oligodendrocytes. The transplanted cells also promoted axonal growth and remyelination, resulting in improvement of motor function ( ). Studies of human iPSCs have likewise produced promising results. In these studies, human iPSCs derived primarily from adult fibroblasts ( ) were transformed into neurospheres or neural stem cells and transplanted into animal models of SCI. The transplanted cells were shown to survive in the recipient animal, differentiate into neurons and glia, grow elongated axons, form synapses with the host cells, produce neurotrophic factors, and engage in remyelination. In summary, human iPSCs transplanted into animal models of SCI can replace neurons, myelination, and neurotrophic factors that are lost to injury, adding to the litany of evidence that advocates for the translation of stem cell therapies to human SCI patients.
As discussed above, the use of iPSCs avoids the ethical challenges of ESCs, but iPSCs are not devoid of limitations. As is the case for ESCs, teratoma formation is also a concern in iPSCs ( ). In fact, in one of the studies discussed above, by Tsuji and colleagues, the investigators categorized their iPSC-derived precursors as either safe or unsafe, prior to engraftment ( ). In contrast to the overall positive results observed in mice that received the safe cells, teratoma formation was found in mice that received the unsafe cells. Teratoma formation would serve as a major hurdle to translating iPSCs to clinical use. Thus, alternative protocols for producing iPSC suitable for clinical use that are less likely to produce teratomas are under investigation ( ). Finally, although the transplant of iPSCs can potentially be autologous rather than allogeneic, they still carry a risk for transplant reaction ( ).
Translation to the bedside
Development of a more accurate model
Since most animal SCI studies conducted to date have been limited to the setting of acute SCI, there is a paucity of evidence indicating whether stem cell transplantation can be successful in chronic SCI patients. Furthermore, most studies have utilized rodent models, where the pathophysiology and long-term functional deficits do not necessarily recapitulate what is found in humans. For example, even after severe injury, rodents are capable of some level of spontaneous recovery ( ). These points raise the question of how well findings from such models will translate to human patients with chronic SCI and have motivated the development of animal models that more faithfully reflect the cellular and functional changes found in human patients. In one study, investigators developed a chronic SCI model with Gottingen-Minnesota minipigs ( ). Using a compression approach, investigators were able to produce stable long-term neurological deficits as well as histopathological changes like those seen in humans. This model has been recently used in a study where iPSC-derived NPCs were grafted 2.5 months after compression. Grafts were found to be capable of surviving in both autologous and syngeneic settings, and capable of differentiating into neurons several months after transplantation ( ). Further studies like these in large animals that more accurately model human SCI will be critical for identifying the cell-based therapies that have the most potential for success in human trials.
Human studies and clinical trials
A few clinical trials examining stem cell transplantation in human SCI patients have been completed, with many others ongoing or planned ( Table 3 ). Many of the completed trials have been early stage and were conducted with small sample sizes, and important lessons are to be gleaned. One early report described case studies on eight SCI patients that received bone marrow–derived stem cells ( ). The investigators employed multiple routes of administration: spinal cord, spinal canal, and intravenous injections. The procedure was overall safe and well tolerated. At follow-up, patients reported improved quality of life, based on the Barthel Index previously devised for use in patients in the context of stroke. In another study, Mendonca and colleagues conducted a phase I, non-randomized, uncontrolled clinical trial with 14 subjects that had complete thoracic or lumbar level SCI ( ). The study participants received bone marrow–derived stem cell transplants and were evaluated for sensory and motor changes 6 months after the procedure. Apart from one post-operative complication (cerebrospinal fluid leak), no patients experienced adverse events, deeming the transplantation safe. Additionally, several of the study patients demonstrated modest improvements in sensory, motor, and urologic function.
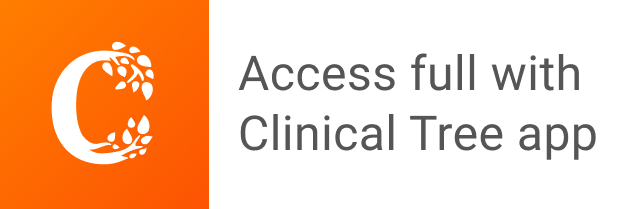