CLINICAL CASE | Horizontal Gaze Palsy With Progressive Scoliosis
Horizontal gaze palsy with progressive scoliosis (HGPPS) is an extremely rare genetic syndrome. It is the result of mutation in the ROBO3 gene, essential for normal axon guidance in many developing neurons, including those of the corticospinal tract and the medial lemniscus. Mutations in this gene are associated with a failure of axonal crossing in certain regions of the brain. Because it is so rare, this syndrome is not likely to be encountered in routine medical practice. Nevertheless, by examining brain images from patients with HGPPS, we have the opportunity to see how brain structure changes when decussation does not occur. Further, by assessing their neurological signs and the results of electrophysiological testing, we have the opportunity to see how mutation can affect the way sensory and motor information is processed by the brain.
A 12-year-old boy with a genetically determined mutation of the ROBO3 gene was examined for somatic sensory, limb motor, and ocular motor functions. Sensory testing did not reveal impairment. He has mild gait instability and a tremor during reaching (intention tremor). He is able to follow a visual stimulus moving vertically with his eyes. He is unable to follow a horizontal moving stimulus. His eyes remain fixated in the forward direction, bilaterally.
Figure 2–1A1 is a midsagittal magnetic resonance imaging (MRI) from a person with HGPPS. Brain tissue is shades of gray (gray matter darker than white matter) and cerebrospinal fluid, black. The bracket is located dorsal to the pons and medulla. Cerebrospinal fluid penetrates into this region on the midline because there is a shallow groove (sulcus). Note that this is not present in the control MRI (Figure 2–1B1). The transverse MRI through the upper medulla (A2) reveals an abnormally flattened appearance in the patient with HGPPS compared with the control brain (B2). A section through the caudal medulla reveals an aberrant deep midline groove (A3, arrow; B3).
Neurophysiological testing was conducted to assess the integrity of the touch and corticospinal motor pathways (see Figure 2–2). To determine the function of the sensory pathway, the skin is electrically stimulated and the overall change in on-going neuronal activity of parietal lobe (ie, EEG) is recorded. Recording the EEG in the region of the somatic sensory cortex in response to electrical stimulation of skin revealed ipsilateral activation, not the customary contralateral activation. To determine the function of the corticospinal tract, transcranial magnetic stimulation (TMS) is used to activate the motor cortex, and the evoked change in muscle activity is recorded. TMS of the motor cortex activated muscles on the ipsilateral side, not the typical contralateral side.
Finally, diffusion tensor imaging (DTI; this is discussed in Box 2–2) was performed on the patient’s MRIs in order to follow the corticospinal tract. This method permits imaging of neural pathways in the brain. Figure 2–1A4 shows the DTI image from a HGPPS patient. There are two parallel pathways. The arrow points to the caudal medulla, where the decussation normally occurs. Furthermore, there are no other decussations along the pathway. B4 is a DTI from a healthy control. The large arrows point to the pyramidal decussation (red) and the smaller arrows, to other brain stem decussations of the pathway. We will learn about these pathways in later chapters.
After reading the explanations below, you should be able to answer the following questions.
1. At what dorsoventral level do the axons of the sensory and motor pathways cross the midline?
2. Is the crossing of all central nervous system axons prevented in this genetic syndrome?
Key neurological signs and corresponding damaged brain structures Dorsal and ventral midline groovesPathways decussate at the midline. In addition to the presence of many structural proteins, decussating axons also provide some physical attachment of the two sides of the brain stem. It is reasoned that without the decussation, or with a reduced number of decussating axons, the two sides of the brain at that spot do not adhere. A space is revealed indirectly because cerebrospinal fluid is present. This is much like seeing cerebrospinal fluid in sulci of the cerebral cortex. Because this syndrome is so rare, there are no histological pathological specimens. Importantly, Figure 2–1A1 shows that the corpus callosum is present in the patient. This indicates that not all decussation is prevented in this genetic syndrome. Callosal neurons use a different mechanism for guiding their axons across the midline than corticospinal tract and medial lemniscal neurons. It is not yet understood how this genetic defect produces scoliosis, which is curving of the vertebral column.
Absence of horizontal eye movementsAs we will learn in Chapter 12, horizontal eye movements are coordinated by neurons that have an axon that decussates in the dorsal pons. Normally, there is an important decussation where the dorsal midline groove is present (Figure 2–1A1). Without this decussation and other circuit change, the eyes are prevented from moving horizontally.
Ipsilateral corticospinal tract and dorsal column–medial lemniscal signalingBoth the corticospinal tract and dorsal column–medial lemniscal system have a decussation in the caudal medulla; the motor decussation is caudal to the somatic sensory decussation. Again, the structurally-abnormal cleft in the ventral medulla points to the absence or fewer decussating axons of the two systems. This is consistent with the results of electrophysiological testing and the DTI for the corticospinal tract. The tremor is probably due to an abnormal circuit between the cortex and the cerebellum. Brain stem neurons transmit signals from the cortex on one side to the cerebellum on the other. This decussation is also prevented in HGPPS.
ReferencesBosley TM, Salih MA, Jen JC, et al. Neurologic features of horizontal gaze palsy and progressive scoliosis with mutations in ROBO3. Neurology. 2005;64(7):1196-1203.
Haller S, Wetzel SG, Lutschg J. Functional MRI, DTI and neurophysiology in horizontal gaze palsy with progressive scoliosis. Neuroradiology. 2008;50(5):453-459.
Jen JC, Chan WM, Bosley TM, et al. Mutations in a human ROBO gene disrupt hindbrain axon pathway crossing and morphogenesis. Science. 2004;304(5676):1509-1513.
Martin J, Friel K, Salimi I, Chakrabarty S. Corticospinal development. In: Squire L, ed. Encyclopedia of Neuroscience. Vol 3. Oxford: Academic Press; 2009:302-214.
FIGURE 2–1
Brain images from patients with horizontal gaze palsy with progressive scoliosis (HGPPS) are shown on the left and images from a healthy person’s brain, on the right. 1. Midsagittal MRI. The bracket marks the region of a midline groove in an HGPPS patient. 2. Transverse MRI sections through the medulla. There is abnormal flattening of the rostral medulla (A2) and a ventral groove in the caudal medulla (A3). 4. Diffusion tensor image (DTI) of the corticospinal tracts. The tracts are shown from about the level of the internal capsule (top) to the caudal medulla (bottom). The patient’s corticospinal tracts (A4) show no crossing fibers, whereas the tract from the healthy person (B4) shows many sites of crossing fibers. The corticospinal tract decussation (red) is at the level of the two large arrows. Other decussations are identified by the smaller arrows. The insets in the bottom right show the imaging planes for parts 1-4. (A1, Reproduced with permission from Bosley TM, Salih MA, Jen JC, et al. Neurologic features of horizontal gaze palsy and progressive scoliosis with mutations in ROBO3. Neurology. 2005;64(7):1196-1203. A2, A3, A4, Reproduced with permission from Haller S, Wetzel SG, and Lutschg J. Functional MRI, DTI, and neurophysiology in horizontal gaze palsy with progressive scoliosis. Neuroradiology. 2008;50(5):453-459. B1, B2, B3, Courtesy of Dr. Joy Hirsch, Columbia University. B4, Reproduced with permission from Poretti A, Boltshauser E, Loenneker T, et al. Diffusion tensor imaging in Joubert syndrome. AJNR. Am J Neuroradiol. 2007;28(10):1929-1933.)

Box 2–1 Anatomical Techniques for Studying the Regional and Microscopic Anatomy of the Human Central Nervous System
There are two principal anatomical methods for studying normal regional human neuroanatomy using postmortem tissue. Myelin stains use dyes that bind to the myelin sheath surrounding axons. Unfortunately, in myelin-stained material, the white matter of the central nervous system stains black and the gray matter stains light. (The terms white matter and gray matter derive from their appearance in fresh tissue.) Cell stains use dyes that bind to components within a neuron’s cell body. Tissues prepared with either a cell stain or a myelin stain have a characteristically different appearance (Figure 2–4A, B). The various staining methods are used to reveal different features of the nervous system’s organization. For example, cell stains are used to characterize the cellular architecture of nuclei and cortical areas, and myelin stains are used to reveal the general topography of brain regions. Myelin staining is also used to reveal the location of damaged axons because, after such damage, the myelin sheath degenerates. This results in unstained tissue that otherwise ought to stain darkly (see Figure 2–5D).
Other staining methods reveal the detailed morphology of neurons—their dendrites, cell body, and axon (Figure 2–4C)—or the presence of specific neuronal chemicals such as neurotransmitters, receptor molecules, or enzymes (see Figure 14–12A). Certain lipophilic dyes that diffuse preferentially along neuronal membranes can be applied directly to a human postmortem brain specimen. This technique allows delineation of some neural connections in the human brain because the axons of neurons at the site of application of the tracer are labeled.
FIGURE 2–3
Groups of brain stem and forebrain neurons have diffuse projections throughout the central nervous system. A. Schematic illustration of the diffuse projection pattern of acetylcholine-containing neurons in the basal nucleus (of Meynert), septal nuclei, and nucleus of the diagonal band (of Broca). Many of the axons projecting to the hippocampal formation course in the fornix (dashed line). B1. Dopamine-containing neurons in the substantia nigra and ventral tegmental area. Yellow marks the location of dopamine neurons in the hypothalamus. B2. Noradrenaline-containing neurons in the locus ceruleus. B3. Serotonin-containing neurons in the raphe nuclei.

FIGURE 2–5
Spinal cord. A. Three-dimensional schematic view of a spinal cord segment showing key spinal cord structures, the circuit for the knee-jerk reflex, and a connection from the corticospinal tract to a motor neuron. B. Myelin-stained transverse section through the cervical spinal cord. The three parts of the spinal gray matter—the dorsal horn, intermediate zone, and ventral horn—are distinguished. The circuits for the reflex and corticospinal tract are also shown. C. MRI through the cervical spinal cord. The red box pinpoints the spinal cord. D. Myelin-stained section through the spinal cord showing unmyelinated regions in the dorsolateral portion of the lateral columns, where the corticospinal tracts are located.

Box 2–2 Magnetic Resonance Imaging Visualizes the Structure and Function of the Living Human Brain
Several radiological techniques are routinely used to image the living human brain. Computerized tomography (CT) produces scans that are images of a single plane, or “slice,” of tissue. The image produced is a computerized reconstruction of the degree to which different tissues absorb transmitted x-rays. Although CT scans are commonly used clinically to reveal intracranial tumors and other pathological changes, the overall level of anatomical resolution is poor. Magnetic resonance imaging (MRI) probes the regional and functional anatomy of the brain in remarkably precise detail. Routine MRI of the nervous system reveals the proton constituents of neural tissues and fluids; most of the protons are contained in water. Protons in different tissue and fluid compartments, when placed in a strong magnetic field, have slightly different properties. MRI takes advantage of such differences to construct an image of brain structure or even function.
MRI relies on the simple property that protons can be made to emit signals that reflect the local tissue environment. Hence, protons in different tissues emit different signals. This is achieved by exciting protons with low levels of energy, which is delivered to the tissue by electromagnetic waves emitted from a coil placed over the tissue while the person is in the MRI scanner. Once excited, protons emit a signal with three components, or parameters, that depend on tissue characteristics. The first parameter is related to proton density (ie, primarily a measure of water content) in the tissue. The second and third parameters are related to proton relaxation times; that is, the times it takes protons to return to the energy state they were in before excitation by electromagnetic waves. The two relaxation times are termed T1 and T2. T1 relaxation time (or spin-lattice relaxation time) is related to the overall tissue environment, and T2 relaxation time (or spin-spin relaxation time), to interactions between protons. When an MRI scan is generated, it can be made to be dominated by one of these parameters. This differential dependence is accomplished by fine-tuning the electromagnetic waves used to excite the tissue. The choice of whether to have an image reflect proton density, T1 relaxation time, or T2 relaxation time depends on the purpose of the image. For example, in T2-weighted images, which are dominated by T2 relaxation time, watery constituents of the brain produce a stronger signal than fatty constituents (eg, white matter); hence, cerebrospinal fluid (CSF) is bright and white matter, dark. These images can be used to distinguish an edematous region of the white matter after stroke, for example, from a normal region.
Four constituents of the central nervous system are distinguished using MRI: (1) cerebrospinal fluid, (2) blood, (3) white matter, and (4) gray matter. The exact appearance of these central nervous system constituents depends on whether the image reflects proton density, T1 relaxation time (Figure 2–6A), or T2 relaxation time (Figure 2–6B). For T1 images, the signals produced by protons in cerebrospinal fluid are weak, and, on this image, cerebrospinal fluid is shaded black. Cerebrospinal fluid in the ventricles and overlying the brain surface, in the subarachnoid space, has the same dark appearance. On T2 images, cerebrospinal fluid appears white, because the signal it generates is strong. In T1 images, protons in blood in arteries and veins produce a strong signal, and these tissue constituents appear white. In T2 images, blood produces a weak signal. This weak signal derives from two factors: tissue motion (ie, normally blood flows and the signals from flowing blood are dispersed and weak) and the presence of hemoglobin, an iron-containing protein that attenuates the MRI signal because of its paramagnetic properties. The gray and white matter are also distinct because their protons emit signals of slightly different strengths. For example, on the T1-weighted image (Figure 2–6A), the white matter appears white and the gray matter appears dark.
Several major deep structures can be seen on the MRI scans in Figure 2–6A, B: the thalamus, the striatum, another component of the basal ganglia termed the lenticular nucleus, and the internal capsule. Figure 2–6D shows a T1-weighted MRI in the sagittal plane, close to the midline. The gyri and cerebrospinal fluid in the sulci look like the drawn image of the medial surface of the brain (Figure 2–6C). The image of the brain stem and cerebellum is a “virtual slice.”
There have been two major advances in the basic MRI technique. Diffusion-weighted MRI (DTI) takes advantage of a component of the MRI signal that depends on the direction of diffusion of water protons within the tissue, which is highly restricted within white matter tracts. This approach can be used to examine fiber pathways in the brain, or tractography. The DTI in Figure 2–7A demonstrates the extensive networks of connections between different regions of the cerebral cortex. The other approach is functional MRI (or fMRI; Figure 2–7B). This technique provides an image of the changes in blood flow–related neural activity in different brain regions. This MRI was obtained while the subject was experiencing the hurt of social exclusion. The image shows that the anterior cingulate gyrus is activated, suggesting it is important in this kind of emotion.
FIGURE 2–6
MRI. T1 (A) and T2 (B) MRI scans produce opposite images. Cerebrospinal fluid appears white on T1-weighted MRI scans and dark on T2-weighted images. T1-weighted images look like a brain slice because gray matter appears dark and white matter appears white. The imaging planes for the two MRI scans are the same, and similar to Figure 2–17. C. Schematic and corresponding T1 midsagittal MRI. (Courtesy of Dr. Neal Rutledge, University of Texas at Austin.)

FIGURE 2–7
A. Diffusion tensor image (DTI) of connections between different areas of cortex. (Courtesy of Dr. Thomas Schultz, Max Planck Institute for Intelligent Systems, Tübingen.) B. Functional magnetic resonance image (fMRI) showing the region in the anterior cingulate gyrus that became active in a subject experiencing the hurt of social exclusion. (From Eisenberger NI, Lieberman MD, Williams KD. Does rejection hurt? An FMRI study of social exclusion. Science. 2003;302[5643]:290-292.)

From a consideration of the regional and functional anatomy of the central nervous system, the principle of functional localization emerges. Each major division of the central nervous system, each lobe of the cortex, and even the gyri within the lobes perform a limited and often unique set of functions. In contrast to most organs of the body—like the heart, stomach, or limb muscles, where their structure helps to predict their function—the gross structure of the brain provides little insight into its overall function, much less the nuances of its role in perception, movement, thought, or emotions. For example, the inferior frontal lobe and the superior parietal lobe look much the same; both have a complex rolling topography of gyri and sulci. Microscopically, they too are remarkably similar, each with neurons organized into six layers. Yet the inferior frontal lobe functions to produce speech and the superior parietal lobe is important in attention. These functional differences come about largely as a result of their connections with other brain regions. The inferior frontal lobe receives information about speech sounds, and connects with brain motor centers, principally those controlling the muscles of the face and mouth. By contrast, the superior parietal lobe receives diverse sensory information, especially visual information, and connects with brain areas important for planning behavior, in particular looking to what interests us. Whereas the logic of functional localization can be understood on the basis of how neural circuits develop specific connections, we have little insight into the logic of why functions are localized where they are.
In addition to the specific connections between structures, there are neural circuits with widespread connections that modulate the actions of neural systems with particular functions. Consider how the quiescent state of a mother’s brain can be mobilized by the sound of her infant’s cry during the night. In an instant, perception is keen, movements are coordinated, and judgments are sound. The neural systems mediating arousal and other generalized functions involve the integrated actions of different parts of the brain stem. Importantly, these regulatory systems use particular neurotransmitters, such as serotonin or dopamine, to exert their actions. These neurotransmitter-specific regulatory systems are also particularly important in human behavioral dysfunction because many of their actions are abnormal in psychiatric disease.
By considering the patterns of neural connections between specific structures, this chapter begins to explain how the various components of the spinal cord and brain acquire their particular sensory, motor, or integrative functions. First, it examines the overall organization of the neural systems for touch and limb position sense and for voluntary movement control. Limb position sense is our ability to detect the location and orientation of our limbs without looking at them. Because these systems travel through all of the major brain divisions to execute their functions, they are excellent for introducing general structure-function relations. Second, the chapter examines the different modulatory systems. Finally, key anatomical and radiological sections through the spinal cord and brain are examined. An understanding of the different neural systems is reinforced by identifying the locations of these systems in the central nervous system. Knowledge of the location of nuclei and tracts in these anatomical sections is important not only for understanding neuroanatomy but also for learning to identify brain structure on radiological images.
The Dorsal Column–Medial Lemniscal System and Corticospinal Tract Have a Component at Each Level of the Neuraxis
The principal pathway for touch and limb position sense, the dorsal column–medial lemniscal system, and the key pathway for voluntary movement, the corticospinal tract, each have a longitudinal organization, spanning virtually the entire neuraxis. These two pathways are good examples of how particular patterns of connections between structures at different levels of the neuraxis produce a circuit with a limited number of functions. This does not mean that there are no other neural systems for these body senses and control functions. Indeed, many systems work together for even the simplest perceptions and movements. Analyzing the basic functions of circuits in isolation, as we are here, provides a starting point for understanding functional neuroanatomy.
The dorsal column–medial lemniscal system is termed an ascending pathway because it brings information from sensory receptors in the periphery to lower levels of the central nervous system, such as the brain stem, and then to higher levels, such as the thalamus and cerebral cortex. In contrast, the corticospinal tract, a descending pathway, carries information from the cerebral cortex to a lower level of the central nervous system, the spinal cord.
The dorsal column–medial lemniscal system (Figure 2–2A) consists of a three-neuron circuit that links the periphery with the cerebral cortex. Even though there are minimally three neurons in this circuit, many thousands of neurons at each level are typically engaged during normal tactile experiences. Each of the neurons in the figure stands for many hundred or thousands. The first neurons in the circuit are the dorsal root ganglion neurons, which translate stimulus energy into neural signals and transmit this information directly to the spinal cord and brain stem. This component of the system is a fast transmission line that is visible on the dorsal surface of the spinal cord as the dorsal column (see Figure 2–5B).
The first synapse is made in the dorsal column nucleus, a relay nucleus in the medulla. A relay nucleus processes incoming signals and transmits this information to the next component of the circuit. The cell bodies of the second neurons in the pathway are located in the dorsal column nucleus. The axons of these second-order neurons cross the midline, or decussate. Because of this decussation, sensory information from one side of the body is processed by the opposite side of the brain. Most sensory (and motor) pathways decussate at some point along their course. While we understand the molecular mechanisms of how some axons cross the midline and others do not, surprisingly, we do not know the reason why particular neural systems decussate. The case presented in this chapter describes the loss of decussation of the dorsal column-medial lemniscal system due to a genetic defect.
After crossing the midline, the axons ascend in the brain stem tract, the medial lemniscus, to synapse in a particular relay nucleus in the thalamus. From here, the third-order neurons send their axons through the white matter underlying the cortex, in the internal capsule. These axons synapse on neurons in the primary somatic sensory cortex, which is located in the postcentral gyrus of the parietal lobe (Figure 2–2A). Each sensory system has a primary cortical area and several higher-order areas. The primary area processes basic sensory information, and the higher-order areas participate in the elaboration of sensory processing leading to perception. Damage to this system, more commonly at the spinal level, makes fine tactile discriminations difficult and impairs our limb position sense.
Axons of the corticospinal tract descend from the cerebral cortex to terminate on motor neurons in the spinal cord (Figure 2–2B). In contrast to the dorsal column–medial lemniscal system, in which fast transmission lines are interrupted by series of synapses in relay nuclei, the corticospinal tract consists of single neurons that link the cortex directly with the spinal cord. The cell bodies of many corticospinal tract neurons are located in the primary motor cortex on the precentral gyrus of the frontal lobe, just rostral to the primary somatic sensory cortex. The axons of these neurons leave the motor cortex and travel down in the internal capsule, near the thalamic axons transmitting information to the somatic sensory cortex.
The corticospinal tract emerges from beneath the cerebral hemisphere to course ventrally within the brain stem. In the medulla, the corticospinal axons form the pyramid, a prominent landmark on the ventral surface. In the caudal medulla, most corticospinal axons decussate (pyramidal, or motor, decussation). In the case study, a genetic impairment resulted in the loss of the pyramidal decussation (Figure 2-1A4). The corticospinal tract axons descend into the spinal cord, where they travel within the white matter before terminating on motor neurons in the gray matter. These motor neurons innervate skeletal muscle; they are sometimes termed lower motor neurons. Motor cortex neurons that give rise to the corticospinal tract are often termed upper motor neurons. Patients with corticospinal tract damage, commonly caused by interruption of the blood supply to the internal capsule or a spinal cord injury, demonstrate a range of impairment, depending on the amount of damage, ranging from impaired fine motor skills and muscle weakness to paralysis. Following these pathways shows how many different brain regions are recruited into action for simple sensory and motor functions.
The Modulatory Systems of the Brain Have Diffuse Connections and Use Different Neurotransmitters
Specificity of neural connections characterizes the somatic sensory and motor pathways. The dorsal column–medial lemniscal system can mediate our sense of touch because it specifically connects touch receptors in the skin with a particular region of the cerebral cortex. Similarly, the corticospinal tract’s role in controlling movement is conferred by its particular connections with motor circuits in the spinal cord. Several major exceptions exist in which systems of neurons have more widespread projections; and in each case these systems are thought to serve more generalized functions, including motivation, arousal, and facilitation of learning and memory. The cell bodies of diffuse-projecting neurons are located throughout the brain stem, diencephalon, and basal forebrain; some are clustered into distinct nuclei and others are scattered. They terminate throughout all divisions of the central nervous system.
Four systems of diffuse-projecting neurons are highlighted here because of their importance in the sensory, motor, and integrative systems examined in subsequent chapters. Each system uses a different neurotransmitter: acetylcholine, dopamine, noradrenalin (norepinephrine), or serotonin. Many of the neurons that use one of these neurotransmitters also contain other neuroactive compounds, such as peptides, that are released at the synapse at the same time. Dysfunction of these systems occurs in many psychiatric and neurological diseases.
The axons of acetylcholine-containing neurons in the basal forebrain (ie, at the base of the cerebral hemispheres) and several other sites, including the lateral hypothalamus, project throughout the cerebral cortex and hippocampal formation (Figure 2–3A). Acetylcholine augments the excitability of cortical neurons, especially in association areas. In Alzheimer disease, a neurological disease in which individuals lose memories and cognitive functions, these cholinergic neurons degenerate. There are also cholinergic neurons in the pedunculopontine nucleus, which is located in the pons. These cholinergic neurons are implicated in disordered movement control in Parkinson disease.
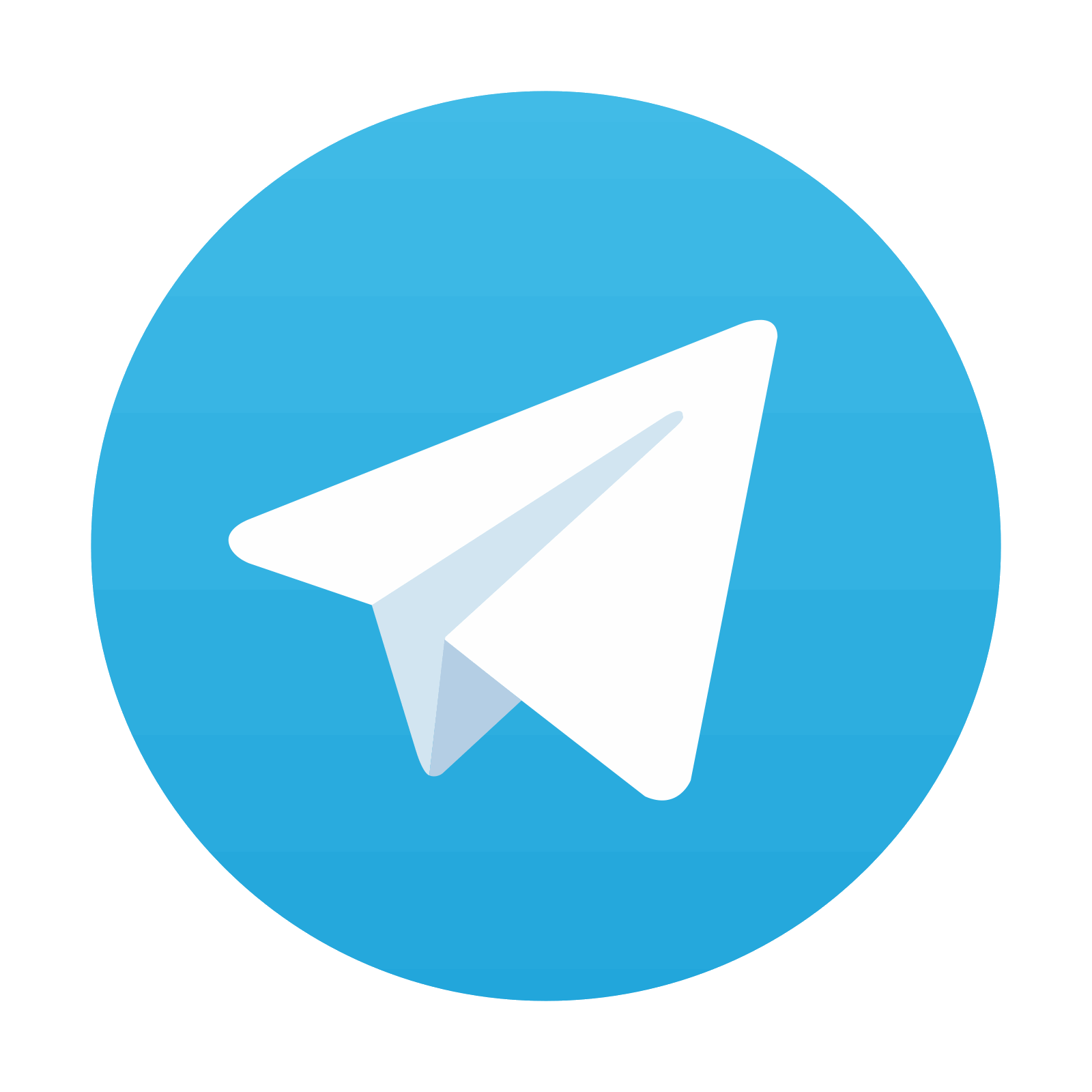
Stay updated, free articles. Join our Telegram channel
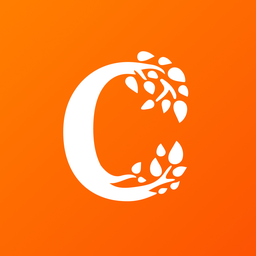
Full access? Get Clinical Tree
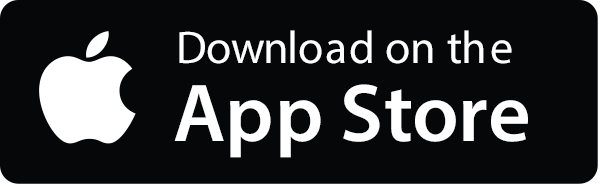
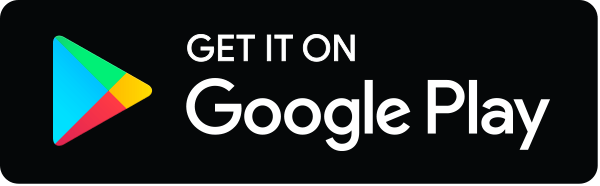