Structural Imaging in Chemosensory Dysfunction
Summary
Structural imaging in chemosensory dysfunction can visualize the morphological anatomy and pathology of the entire chemosensory pathway, from the oral and nasal mucosa and adjacent structures (e.g., paranasal sinuses), along the particular cranial nerves, and through the respective ganglia to the brain. While some of these structures display only subtle and mainly size-related changes during life, the olfactory system contains highly plastic structures. For example, research indicates that smell deficits leading to a reduced sensory input result in structural changes at the level of the olfactory bulb. Therefore, certain cases require highly specific and optimized imaging with quantitative image analysis. However, in most patients, structural imaging in chemosensory dysfunction is a comparatively nonspecific diagnostic tool used mainly to exclude malignancy in the brain and the head and neck region. Clinical symptoms prompting imaging of the chemosensory system typically occur only if both sides of the respective structures are affected, which is more frequent in olfaction than in gustation. If only one side is affected, most patients will neither experience any chemosensory dysfunction nor be able to lateralize the loss of chemosensory input.
This chapter discusses some of the radiological basics for the assessment of chemosensory structures, results of quantitative imaging, and major pathologies in which imaging is clinically relevant.
Imaging Technique
Magnetic resonance imaging is the modality of choice for imaging chemosensory dysfunction.
Technical Considerations: Magnetic Resonance Imaging
Field Strength
Magnetic resonance imaging (MRI) is the major imaging modality used to visualize the anatomy and pathology of chemosensory dysfunction, mainly because of its inherent high soft tissue contrast. It relies on signals from hydrogen atoms. The number of hydrogen atoms contributing to the MRI signal is increased at higher field strengths. Therefore, it may seem that small structures, such as the olfactory bulb (OB) ( Fig. 17.1 ) and the olfactory tract (OT), are best visualized at 3 to 7 T. However, certain artifacts that increase with field strength reduce the applicability of these systems. For example, the main parts of the OT are not easily visualized at a field strength at or above 3 T, as so-called susceptibility artifacts, occurring at the posterior frontobasis between cerebrospinal fluid (CSF) and the air-filled paranasal sinuses, may deteriorate signal ( Fig. 17.2 ). Furthermore, these systems are still not available everywhere. Therefore, the imaging technique was optimized at 1.5 T, using advanced receiver-coil techniques or selected imaging sequences. The techniques discussed herein were performed at 1.5 T, which remains the reference standard for many applications. Most of the images in this chapter were acquired at 1.5 T.

Receiver Coils
When MRI was first used to visualize the OB, mostly small-loop coils were employed, which were placed on the nasion, and enabled comparatively high-resolution images of the OB to be obtained.1 Owing to the limited field of view (“depth penetration”) of these coils, the OT and posterior parts of the olfactory system could not be visualized, and standard head coils were applied, allowing imaging of the whole brain at somewhat lower spatial resolution. New head coils (e.g., multichannel head array coils) allow similar or even better spatial resolution than small-loop coils and provide information from the entire brain.

Sequence Techniques
Basically, two different tissue characteristics may be assessed: the T1 time, using T1-weighted sequences; and the T2 time, using T2-weighted sequences. Different sequence types (mainly turbo spin echo [TSE], constructive interference in steady-state precession [CISS], turbo fast low-angle shot) are capable of generating different contrasts between tissues. Additionally, and independent of the desired tissue contrast, sequences may be optimized for three-dimensional (3D) imaging or two-dimensional (2D) imaging.
For high-resolution imaging, 2D data acquisition is preferably performed using TSE techniques, which generate a voxel size of 0.405 mm3. A voxel is a 3D picture element (volume element) consisting of the slice thickness (mm) multiplied by the in-plane resolution (mm × mm). With such small voxels, accurate volumetry of the OB is feasible. To accurately assess this, 2D TSE sequences should be acquired in coronal slice orientation (perpendicular to the frontobasis; maximum slice thickness 2 mm) covering the entire frontal skull base and the pituitary gland. To visualize pathology along the OB or within the Meckel cave, a sequence with stronger T2-weighting (e.g., CISS2) appears to be advantageous. This technique generates bright signals from CSF and very low signal intensities from all other tissues and structures (i.e., high contrast for the sensitive visualization of structures surrounded by CSF [ Fig. 17.3 ]). The disadvantage of this sequence is the poor contrast among nerves, brain, air, and bone.
If the entire afferent olfactory or gustatory system, including nasal and oral cavity and posterior structures, needs to be investigated, 3D imaging with isotropic voxels (which reveal identical lengths of the margins of every voxel in all three spatial orientations) covering the entire cranium is preferred. The acquired datasets allow for multi-planar reformation in any orientation and may be acquired before and after intravenous administration of contrast material. For such large volume acquisitions, imaging is preferably performed at 3 T to keep the acquisition time short whilst providing adequate spatial resolution.

For clinical purposes, and mainly to exclude frontal, prepontine, or intra-axial tumors or infections, the imaging protocol includes T1-weighted 2D imaging before and after intravenous administration of contrast material. Imaging should be performed using axial, coronal, and sagittal planes to provide sufficient information for preoperative planning. The use of fat suppression techniques improves the delineation of extracranial tumors growing along cranial nerves and invading subcutaneous fat. For tumor detection in the head and neck region, so-called TIRM (turbo inversion recovery magnitude) sequences with strong fat suppression (inversion time above 160 milliseconds) are preferably performed, as these imaging techniques are highly sensitive at detecting pathologies surrounded by normal soft tissues, especially fat.
s. Kap. Table 17.1 shows important technical parameters of recommended sequence designs.
New Developments
As the signal in MRI relies on hydrogen, the contrast between cortical bone and air is low. New techniques, such as sequences delivering ultrashort echo times, allow for enhanced contrast between air and bone, and may be applied to MRI of the paranasal sinuses in the near future.
Limitations
Current limitations of MRI in structural imaging of chemosensory dysfunction are related to the size of the object and the contrast of the object in relation to its surroundings. Higher field strengths are pushing the limits, but cellular imaging, for example, is only possible using labeled cells. Imaging of single neurons or even groups of cells is currently not possible with MRI without labeling.
Patient-related contraindications of MRI are continuously decreasing. While cardiac pacemakers have been an absolute contraindication, some institutes are now performing MRI in patients with such devices under dedicated surveillance. Continuing contraindications are ferromagnetic foreign bodies in regions where their movement may destroy structures, especially vessels or neural structures.
The use of gadolinium-based contrast agents continues to be considered very safe, even in patients suffering from severe renal insufficiency (glomerular filtration rate < 30 mL/min/1.73 m2).
Technical Considerations: Computed Tomography
Image Acquisition
Computed tomography (CT) imaging contrast is dependent on the electron density of the visualized tissues. Therefore, soft tissue contrast is low compared with MRI, and some structures are not easily visualized (e.g., the OB). However, CT imaging of bone- and air-filled structures reveals a high difference in electron density and can provide sufficient diagnostic information in some cases of chemosensory dysfunction. Destruction of bones related to trauma or infiltration and replacement of air in cranial cavities are the main pathologies visualized by CT ( Fig. 17.4 ). Additionally, the visualization of the foramina where the cranial nerves penetrate the skull base is a major indication for CT in chemo sensory imaging. For this indication, CT is only limited by spatial resolution (e.g., the foramina of the cribriform plate are not visualized in standard CT imaging). Typically, a 3D dataset of the skull is acquired and reformatted in three orthogonal imaging planes. Data acquisition is mainly performed in an axial imaging plane, but also may be performed or supplemented in a coronal imaging plane. This depends on the clinical question: while trauma and tumor questions are most often answered with axial imaging, pathology of the paranasal sinuses is mainly assessed using coronal imaging. Additionally, artifacts related to metal-based dental implants, which may impair image quality, have to be taken into consideration when planning image acquisition.

Nowadays, CT is acquired as a true (isotropic) 3D dataset revealing a voxel size between 0.6 mm × 0.6 mm × 0.6 mm (voxel size: 0.216 mm3) and 1 mm × 1 mm × 1 mm (voxel size: 1 mm3), and the desired imaging planes are reformatted out of this dataset.
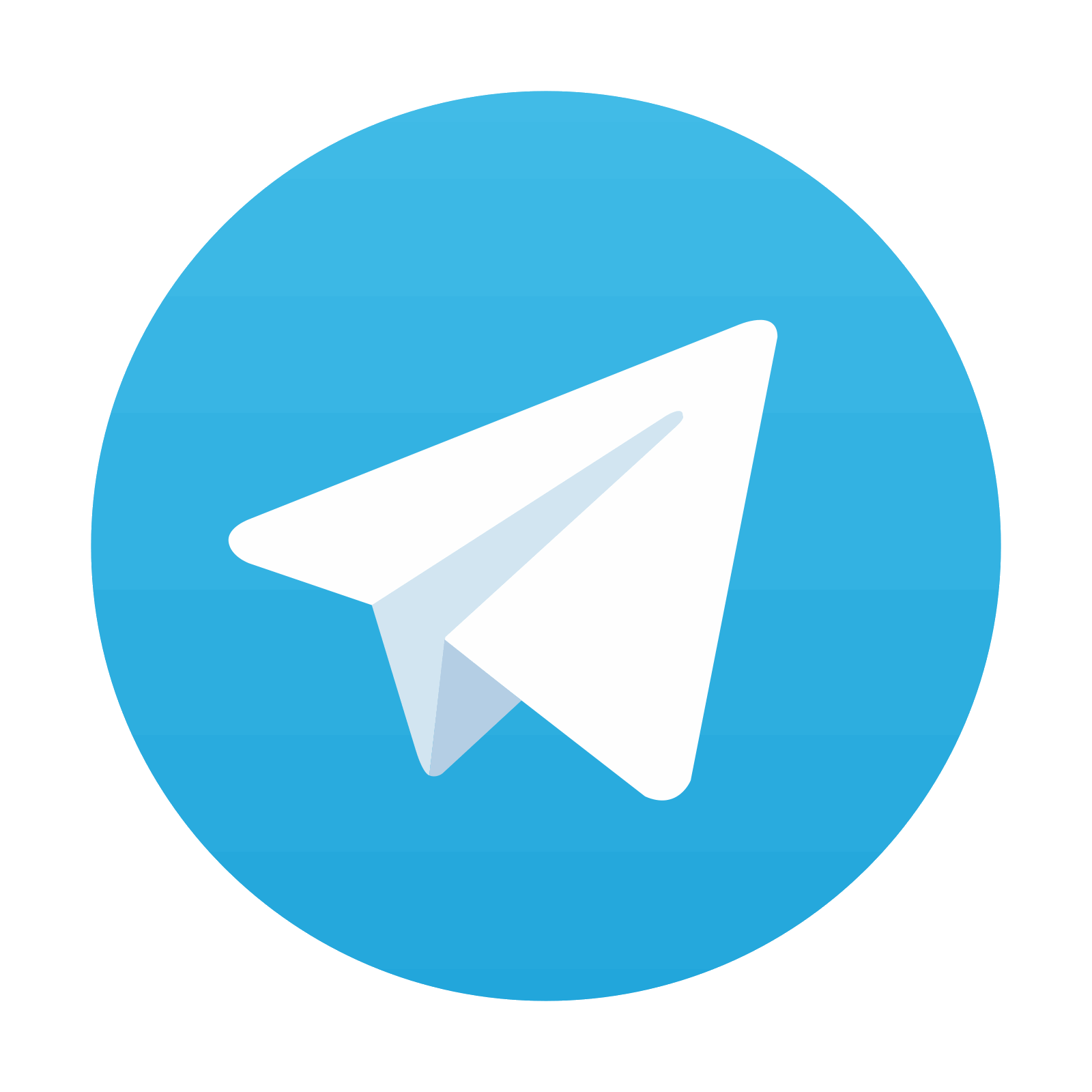
Stay updated, free articles. Join our Telegram channel
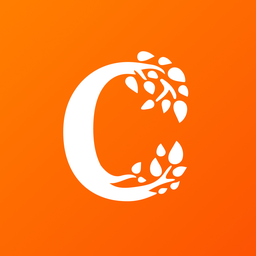
Full access? Get Clinical Tree
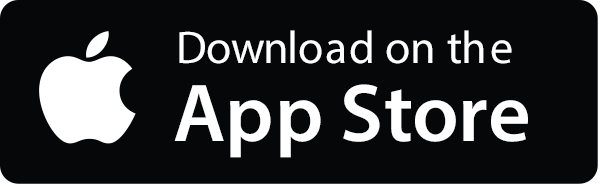
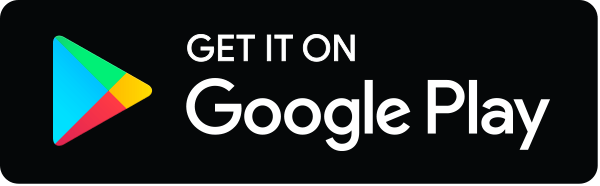
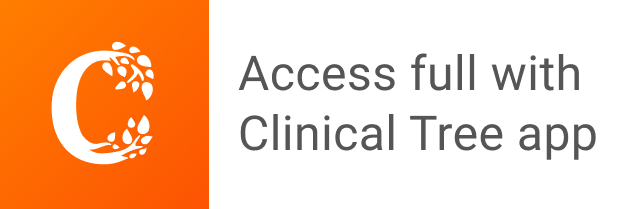