List of abbreviations
ADL
activity of daily living
AIS
ASIA impairment scale
ARTIC
advance robotic therapy integrated centers
ASIA
American Spinal Injury Association
CNS
central nervous system
CPG
central pattern generator
FES
functional electrical stimulation
SCI
spinal cord injury
Introduction
The incorporation of robotics in the field of neurorehabilitation is taking place rapidly, both in research and in its clinical applications, and is presented as a very promising tool that is changing therapeutic paradigms. In the late 1980s and early 1990s, basic research findings constituted a major change in therapeutic intervention in neurorehabilitation. One of most relevant was that in experimental models with cats subjected to a spinal cord injury (SCI), the subsequent training of the locomotor function applied to them offered good results. In fact, it was shown that these cats with SCI walked effectively when placed on a treadmill with partial weight support ( ). The suggested mechanism is the activation of the basic neuronal circuitries sufficient to generate efficient stepping patterns and independent standing. Indeed, the operations underlying the elaboration of motor patterns for walking and standing are essentially achieved by the neuronal networks embedded within the lumbosacral segments of the spinal cord ( ). These findings led to the concept of spinal learning via activity-dependent plasticity. Following this concept, it was found that locomotor activity can be activated in patients with severe SCI via passive activation of the legs on a treadmill ( ). Synchronous reciprocal movements of both legs, simulating normal walking are required to activate the locomotor centers in the spinal cord. The repetitive and simultaneous activation of certain sensory and motor pathways with task-specific training can select and reinforce those spinal circuits improving the ability to perform the practiced movement successfully. Thus, functional rehabilitation (i.e., walking) had to be intensive and task-oriented. Intensive and task-oriented are the two are the pillars of the motor learning neuroplasticity-based neurorehabilitation concepts that also justify the development of robotic therapy ( ; ).
Although these interventions appear promising, in order to translate them into clinical practice in humans, a great effort is needed to standardize the assessments of the therapies applied ( ). Gait training using partial weight bearing systems on treadmills in patients who had suffered a stroke or SCI was extended in the early 1990s following motor learning principles. This therapy initially presented high costs in terms of personnel and effort, as it required the participation of at least two physiotherapists to mobilize the paralyzed lower extremities of the patient with the intention of reproducing the treadmill walking cycle ( ). The great effort that this activity demanded from the physiotherapists limited the duration of the treatment sessions. This limitation led to the idea that a robotic device could serve as an alternative to manual treatment and that such a device could cover the demands of functional training ( ). This led to the first robotic systems for walking training with weight suspension on treadmills.
These robotic assistive devices enable to start a functional and task-oriented training as soon as possible after the injury and allow an intensive application of adequate afferent feedback and a high number of repetitions of functional movements ( ).
Furthermore, the outcome of rehabilitation is better if the patient is more motivated and involved in the treatment ( ). All these without forgetting one of the most evident shortcomings of conventional systems, which is the need to incorporate sensors that provide objective variables of the patient’s condition or of the execution of the task, need to be trained. These issues are satisfactorily addressed by robotic devices. This therapy can be applied alone or in combination with other new technologies such as functional electrical stimulation (FES) or virtual reality.
Robotic therapy has experienced a huge boom in the last 15 years. In fact, different clinical guidelines approved its use as a complementary element to conventional therapy in the rehabilitation of patients with upper limb deficits after suffering a stroke ( ). Robotic devices are appropriately adapted to the need to assist limb movements based on their ability to perform simple, repetitive tasks in a consistent manner that facilitates functional recovery and adaptive plasticity ( ). There are two main categories: distal end effector devices and exoskeleton-type devices. Distal end effectors were the first to appear and are characterized by the fact that they use a single distal point of contact to guide the movement of the entire limb. In the upper extremity, it can make contact in the hand or forearm, facilitating the movements of the elbow and shoulder. They produce combined movements being difficult to isolate pure simple movements. The operation of exoskeletons is different. They are structures located in parallel to the different parts of the extremities with more than one point of interaction with the person. They provide direct control over each segment of the limb by incorporating individualized motors, also called actuators, which coincide with the anatomical axis of each joint. Thus, each actuator triggers the movement of each joint on which it is located. The design of exoskeletons seems to be more suitable than that of distal effector systems to achieve large joint paths ( ).
In this chapter, we will focus on upper limb robots, stationary and ambulatory lower limb exoskeletons.
Upper limb robots
Cervical SCI can result in partial or complete tetraplegia. Each small improvement in motor control of the upper extremity can translate to significant ameliorations in function and increases independence for the individual. As mentioned above, this type of therapy offers new possibilities in the rehabilitation not only for the lower limbs but also for the upper limbs. The robotic devices allow the application of high-intensity sessions during longer periods of time, remaining invariable certain physical parameters such as speed, strength, or precision ( ; ). There is evidence that suggests task-based therapy specifically designed to deal with lost abilities produce better results than resistance strengthening exercises ( ). This task should be performed by the patient as far as possible. That’s why the devices should be equipped with a controller that provides the least assistance needed to accomplish the movement (assist as needed) and reproducible treatment protocols.
Some studies point out that by focusing the improvement of robotic therapy more on the proximal recovery of the upper limb (shoulder and elbow), it does not translate into improvement of the functional ability that depends on hand control. However, the best results seem to be found by adding the application of both types of therapies ( ). Despite the low number of studies, results from these studies suggest that robotic training protocols are feasible and well tolerated and have a positive impact on improving arm and hand functions in selected patients with cervical SCI, but the results must be interpreted with caution ( ). In any case, studies with larger samples are needed, especially those that analyze the distal region of the upper limb, in order to have solid conclusions about the effectiveness of these devices.
Most of the current devices include a virtual reality module with visual or haptic feedback to improve sensory feedback, as well as patient motivation and engagement. They also have the capability to obtain movement kinematics that can provide precise information about movement quality that otherwise is not included in functional assessments ( ).
Although there is a number of different robotic devices currently used for neurorehabilitation of the upper extremities following SCI ( Fig. 1 ), we will now focus on the most commonly used:

MIT MANUS
It was designed to provide high-intensity and reproducible upper limb rehabilitation in adults and older children. This modular distal effector system consists of a series of proximal and distal components that can be used individually or together for upper extremity training. It comprises two modules and 5 degrees of freedom, two for elbow and forearm motion, and three for wrist motion and allows patients to perform reaching movements in horizontal plane. The robot can move, guide, or perturb the movement of a patient’s upper limb and record quantities, such as position, velocity, and force. The operating paradigm is the so-called “assist as needed.” Thanks to motion sensors, the mobility of the joint segments can always be monitored. The patient–robot interface consists of video games for elbow, shoulder, and wrist exercises that can be used to increase the quality of therapy sessions as well as keep the user engaged ( ). It has initially been used in the rehabilitation of the upper limb of stroke patients, proving effective in the sub-acute and chronic phases by reducing motor deficits, improving function and bringing about a lasting change ( ; ). The commercialized version of MIT-MANUS, INMOTION (Bionik Laboratories Corp., Toronto, Canada), has been used in patients with SCI to a limited extent although one study demonstrated that after a training protocol, significant improvements in quality of movement were found with no changes in upper extremity strength, pain, or spasticity ( ).
ReoGo
The ReoGo system (Motorika Medical, Caesarea, Israel) is a stationary fixed based end-effector arm rehabilitation robot, which facilitates the mobilization of the upper limb on a support that allows a wide range of movements in the 3 dimensions of space. The Reo-Go allows for movements at the shoulder, elbow, and wrist. It also uses a real-time visual feedback monitor to display games for the subject to perform. Although it has primarily been used for stroke patients, it has also been applied in SCI. Reo-Go was incorporated into an acute incomplete SCI patient therapy protocol. The subject demonstrated remarkable improvements in muscle strength, active range of motion and functional assessment ( ).
Armeo
Armeo devices (Hocoma AG, Volketswil, Switzerland) were the first unilateral upper extremity exoskeletons marketed for upper limb rehabilitation. This range of devices includes the Armeo Power for the most affected patients, the Armeo Spring, the Armeo Spring for children and the Armeo Senso for those less affected.
The Armeo system is a well-documented device and is the only device shown to offer better functional results after stroke compared to traditional therapy. Two studies showed the utility of this device for upper limbs in SCI subjects focusing on the potential of these devices in performing upper limb assessment ( ; ). The Armeo Power is one of the most advanced active exoskeletons for upper extremity rehabilitation. It is based on the ARMin device, which consists of an exoskeleton covering the upper limb and allowing anthropometric adaptations. It provides support for the weight of the patient’s upper limb and features different modes of use, such as mobilization mode, 2D and 3D games and functional training of daily life activities. ARMin provides three actuated degrees of freedom for the shoulder and one for the elbow joint. It offers three different therapy modes: the movement therapy, the game therapy and the ADL (activity of daily living) training mode. Like the MIT-MANUS, Armeo Power uses an “assist as needed” mode of operation, allowing the clinician to adapt the difficulty of the task to the degree of recovery. There are studies that demonstrate its usefulness in patients with SCI ( ).An earlier version is the Armeo Spring that manages to cover the shoulder and elbow, and also works the wrist flexo-extension and manual gripping. It is a passive exoskeleton ( Fig. 2 ). It works through a system of springs that eliminate the weight of the body as an enabler instead of using motors to assist movement as the Armeo Power does. Both feature a monitor with motivational games to encourage repetitive movements. The software allows the clinician to select the task and its degree of difficulty by defining the required joint path and the rhythm of the selected game.

Other devices
There are other devices on the market such as the DIEGO (Tyromotion, Graz, Austria) which uses a wiring system to support and mobilize the limb, the Bi-Manu-Track (RehaStim, Germany) which facilitates the treatment of both upper limbs simultaneously. There are devices that focus on the individual mobility of each finger but allow practice in gripping by controlling the performance of each finger. This would be the case of AMADEO (Tyromotion, Graz, Austria), HAND-CARE 2 and RUTGERS-MASTER II (Rutgers University, USA), although the latter excludes the treatment of the fifth finger. It is not common but in some cases two robotic devices have been used in combination, such as the Armeo Power and the Amadeo, using the first for the shoulder, elbow, and carpal and the second for the shoulder, elbow, and carpal.
Stationary lower limb robots
Thoracic and lower SCIs can result in partial to complete paralysis of the lower extremities. Independent mobility for many can only be achieved at a wheelchair level, although walking oftentimes remains a priority ( ). Lower limb robots have emerged as potential upright mobility devices for those with lower limb paralysis. Locomotor training focuses on retraining the motor function via plastic change ( ; ), and the neurophysiological mechanism underlying the restoration of human restoration after SCI involve enhancing the afferent input to the spinal cord and activating CPG (central pattern generator) embedded within the lumbosacral spinal cord ( ). Plastic changes can be induced in both the spinal cord level and sensory motor cortex via intensive locomotor training, mainly in incomplete SCI subjects ( ).
As it has been previously referred, to manually replicate a normal walking pattern with the patient in body weight-supported on a treadmill two or three therapists are needed to control and move lower limbs. This is a strenuous and exhausting task for therapist, so sophisticated automated electromechanical devices have been developed ( ) that offers several advantages, including the ability to increase the intensity and total duration of training while maintaining a physiological gait pattern.
As in the case of the upper limbs, in the lower limb robots we also find, depending on their structure, distal effectors and exoskeletons ( Table 1 ). Among lower limb robotic exoskeletons, we can distinguish the stationary and the ambulatory ones. In this section we will discuss the stationary ones, and the ambulatory will be analyzed in the following section.
Lower limb robots | ||
---|---|---|
Stationary robots | Ambulatory exoskeletons | |
Distal effector | Stationary exoskeletons | Ex: Ekso Bionics, Rewalk, Indego, etc. |
Ex: Gait Trainer | Ex: Lokomat |
End effector devices
End-effector-based systems work like conventional elliptical trainers: the subject’s feet are strapped to two footplates moving along a gait-like trajectory, as in an elliptical trainer, moving the entire lower limb. They work based on a constraint at the distal end of the kinetic chain that specifies the trajectory there and the proximal joints can simply move as the body geometry and articulations dictate. The footplates generate the stance and swing phases in most instances with symmetric motion. The main difference compared with exoskeletons with a treadmill is that the feet are always in contact with the moving platform, simulating the gait phases but not necessarily generating true swing and stance phases. The trajectories of the footplates, as well as the vertical and horizontal movements of the center of mass, are programmable. The end-effector design lends itself to gait retraining and star climbing ( ). Examples of end-effector devices include Gait Trainer GT1 (Reha-Stim, Berlin, Germany), G-EO (Reha Technologies, Switzerland) and Lokohelp. In relation to SCI patients, 3-dimensional data were obtained with Lokomat and G-EO. Their kinematic data were compared when devices were used by SCI or traumatic brain injury patients. The results confirmed a more controlled and repetitive gait pattern when using Lokomat and the G-EO system provided a gait pattern that had more variability of motion for the hips and knees, with slightly reduced knee motion, and the gait pattern differed slightly from that observed during overground walking ( ).
Stationary exoskeletons
Stationary exoskeletons have a device that surrounds the patient’s legs, which may be suspended from an overhead guide rail, supported by a metal frame on wheels, or the exoskeleton can even be directly supported by a mobile robot. They are usually connected directly to the ground through a rigid frame or bolted to a wall, enhancing and ensuring total safety. Stationary exoskeletons can have a large and powerful motors and controllers. They often involve walking on a treadmill. These devices are less complex in their engineering requirements and more stable and safer than ambulatory exoskeletons that allow overground walking due to the elimination of fall risk. They are less accommodating of individual gait variations, such as changes of speed or direction. This group of stationary exoskeletons includes the Lokomat, Walk-Trainer, LOPES or ReoAmbulator.
The Lokomat (Hocoma AG, Volketswil, Switzerland) is the most clinically implanted and studied robot on the market. The Lokomat is a bilaterally driven gait orthosis that is used in conjunction with a body support system ( ). It is essentially a robotic implementation of the treadmill walking training system with partial weight support and manual mobilization of the patient by physiotherapists. This system consists of a treadmill, a partial weight support system and a bilateral exoskeleton that provides action on the hips and knees with the ankle being passively supported by a spring to facilitate dorsiflexion of the swing phase of walking ( ). The Lokomat moves the patient legs through the gait cycle mainly in the sagittal plane ( Fig. 3 ). The device’s hip and knee are actuated by linear drives integrated into an exoskeleton structure. There is no actuator on the ankle and dorsal flexion during the swing phase is achieved passively by means of springs. The lower limb motion can be controlled with highly repeatable predefined hip and knee joint trajectories on the basis of a conventional position control strategy. The exoskeleton is fixed to the rigid frame of the body weight support system and the patient is fixed to the exoskeleton with straps around the waist, thighs and shanks.
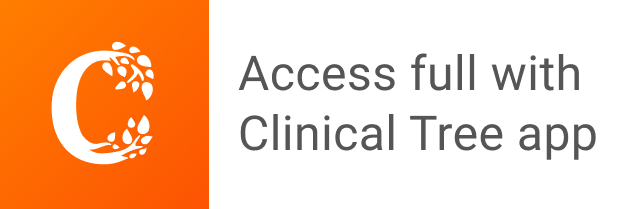