Schematic diagram of the nuclei and pathways of the basal ganglia. The nigrostriatal pathway from the substantia nigra pars compacta (SNpc) to the striatum is dopaminergic. In PD the results of dopamine deficiency include increased neuronal activity in the subthalamic nucleus (STN) and GPi, with irregular and bursting neuronal firing patterns. Increased synchrony is found among sensorimotor networks, which may contribute to PD motor signs.
The subthalamic nucleus (STN) is an important nucleus in the functional organization of the basal ganglia.1,2 The STN receives direct excitatory inputs from almost all parts of the cortex and exerts a powerful excitatory effect on its target nuclei, globus pallidus internus (GPi), globus pallidus externus (GPe), SNpr, SNpc, and the pedunculopontine nucleus (PPN).1,3–6 Through fast-conducting pathways it is hypothesized the STN relays the overall cortical command (template) over the pallida, upon which the focusing of movement takes place through the slower, integrated striato-pallidal pathways. Information processing through the basal ganglia is believed to occur through functionally distinct circuits that are components of a network connecting cortical and subcortical structures. The circuits carrying sensorimotor versus limbic or associative information are largely segregated in different regions of each nucleus. Within the sensorimotor regions of each nucleus, there is somatotopic segregation of arm versus leg versus facial representation.7–9
Movement disorders may be thought of as brain arrhythmias: DBS as a brain pacemaker
Loss of dopaminergic input to the striatum results in the appearance of the classical motor signs of PD. Dopamine depletion in the striatum results in an increase in neuronal activity in GPi and STN, which in turn leads to an increased inhibitory output from GPi through thalamocortical and brainstem projections. Mean neuronal firing rates are increased in both STN and GPi in MPTP (1-methyl-4-phenyl-1,2,3,6-tetrahydropyridine) treated primates,2,3,10–13 and in patients with Parkinson’s disease.14,15 Experimental results in MPTP-treated monkeys have shown that increased firing rates in both GPi and STN occur prior to the onset of symptoms.16 Neuronal firing patterns in untreated PD are irregular, with oscillatory and bursting firing modes. In dystonia, firing patterns are also irregular and bursting, but with lower mean firing rates. In tremor, firing patterns in basal ganglia nuclei and the thalamus are oscillatory and may be directly or indirectly correlated to the tremor frequency.17
A current theory is that the DBS system acts as a brain pacemaker. Chronic high-frequency electrical stimulation of the target nucleus probably entrains irregular neuronal firing patterns and desynchronizes pathological hypersynchronization within sensorimotor circuits.18,19
The goal is to place the DBS lead accurately in the center of the sensorimotor region of the target nucleus and/or in proximity to sensorimotor circuits traversing the region. Equally important is to avoid being close to other neighboring nuclei or fiber tracts, the stimulation of which will cause adverse effects.
DBS lead implantation
It is to be emphasized that DBS can be termed local therapy. DBS affects only local circuits and brain regions, unlike medication which affects the whole body by transmission through the bloodstream. The effect of the electrical field produced by DBS falls off with the square of the distance from the electrical source.20 Thus, accurate placement of the DBS lead in sensorimotor regions is critical to the success of the therapy.
DBS implantation is a team-based neurosurgical procedure, led by a neurosurgeon, who should be formally trained in functional, stereotactic neurosurgery. The components of the typical approach to the procedure are initial anatomical targeting, burr hole placement and headstage attachment, intraoperative microelectrode recording (MER), intraoperative microstimulation, and intraoperative testing with stimulation using the DBS lead after it has been placed. The members of the team working with the neurosurgeon are usually a neurologist and/or a neurophysiologist, an anesthesiologist, and support personnel. The role of the neurologist is to assist the neurosurgeon with the MER and to perform neurological testing of the patient during intraoperative microstimulation and DBS test stimulation. The procedure is performed with the patient awake and under local anesthesia only. Image-guided placement of DBS leads, without physiological recording or stimulation, will be covered in Chapter 4.
Anatomical targeting
Precise anatomical targeting requires stereotactic functional techniques to identify the nucleus of interest and to calculate the first microelectrode trajectory, such that it traverses the sensorimotor region and avoids major cortical and subcortical blood vessels. Accurate targeting is the most important aspect of the procedure, upon which the other tasks rely. If the first microelectrode pass is not even in the nucleus of choice, then finding the sensorimotor region within the nucleus is much harder and will require more MER passes to find the target.
A wide range of targeting techniques is currently used. In a recent survey of 36 North American DBS centers,21 most sites used the stereotactic frame (Leksell, CRW, or Compass) for initial targeting and intraoperative electrode guidance, but a few used the frameless stereotactic technique. The frame-based and frameless techniques require preoperative imaging using MRI and/or MRI/CT (computed tomography) fusion techniques for initial anatomical targeting. The frame-based targeting uses fiducial markers on the frame as a guide, and thus the targeting is performed on the day of surgery. The frameless system is an image-guided system that uses fiducial markers on the skull; thus, image-based targeting can be performed the day before surgery.22 A comparison study has shown that the accuracy of DBS lead placement was not significantly different between the frame-based and frameless system.22 Both had an error margin of 3.5 mm from the expected target to the actual location of the electrode on the DBS lead. This error may be significant in these small deep structures, as a small deviation from the planned target could place the electrodes on the DBS lead outside the nucleus (for example, close to the internal capsule) and/or in a cognitive or associative region within the nucleus. Current potential imaging and surgical errors include a 2–3 mm error in MRI targeting, errors in MRI/CT fusion techniques, the potential of brain shift when replacing the mapping electrode with the DBS lead (see below), and the potential brain shift that may occur over time in the operating room due to loss of cerebrospinal fluid (CSF).
Intraoperative microelectrode recording
Studies from ablative procedures such as pallidotomy and from DBS procedures have confirmed that the optimal location of the lesion or DBS lead spans the sensorimotor region of the target nucleus.15,23–27 The sensorimotor regions of the basal ganglia nuclei are anatomically segregated in terms of somatotopy and function and may have quite irregular shapes (Figure 3.2). Sensorimotor circuits mediating simple motor actions are situated in the dorsolateral aspect of the STN, whereas associative and limbic functions appear to be situated in the central aspects and medial pole of the STN, respectively, although there is some overlap among regions (see Figure 3.2a).9,28,29 Due to the small size of the STN, deviations from the sensorimotor region of the nucleus to the medial portion of the nucleus risk affecting associative and/or limbic STN and potentially causing cognitive or psychiatric side effects. The distance between these regions can be as small as 3–4 mm and so, until current steering devices are available, it is especially important to try to place the DBS lead in the center of the sensorimotor region. MER allows precise identification of the sensorimotor region in each nucleus and compensates for any anatomical targeting errors.
The anatomical segregation of function and somatotopy in the STN (a) and GPi (b). Within the STN there is anatomical segregation of simple versus complex movement, eye movement, mood, and cognitive function.
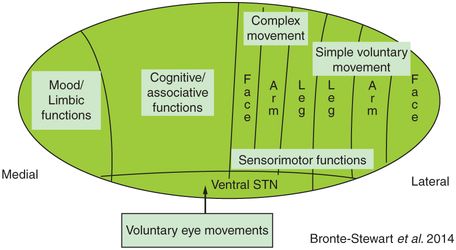
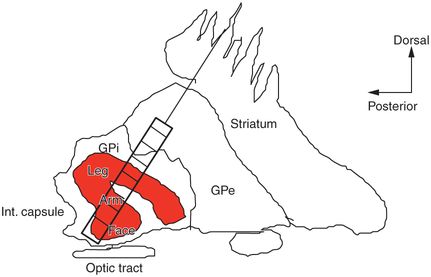
MER techniques vary from institution to institution regarding the use of single neuronal (high-impedance, microelectrode) versus multi-unit (lower impedance, semi-microelectrode) recording and whether one or several microelectrode passes are made to determine the final location for the DBS lead. The North American survey revealed that all centers used some form of intraoperative electrical confirmation of the DBS lead placement, and over 95% used electrophysiological recording.21 Most centers use multipass MER to identify the sensorimotor region, with an average of 2.3 ± 1.4 passes per case. Several studies have shown that the use of MER in addition to anatomical targeting proved to be more precise than surgical targeting based solely on anatomical information provided by a T2-weighted MRI.30–32 However, concern has been expressed that using multiple microelectrode passes to define and map the target structure could increase morbidity and add risk to the patient.33 One large multicenter trial of DBS for PD (268 cases) suggested that microelectrode recording may increase the risk of hemorrhage,34 but this was not found to be a significant cause of hemorrhage in another large single-center study of DBS in 481 cases.35 In our experience, intraoperative MER does not cause morbidity and improves bradykinesia even before placement of the DBS lead.36
MER: method for determining anatomical boundaries and the sensorimotor region
A 0.5–1 megaOhm platinum–iridium microelectrode is inserted into a guide tube (or cannula) lined with Teflon to reduce microphonics. The entire microelectrode/guide tube assembly is carried by a motorized microdrive mounted on a head stage. The microelectrode is advanced within its guide tube to a depth of 15 mm above the target. It is then slowly advanced beyond the guide tube as the MER takes place to below the target depth at the nucleus. A scribe notates the activity encountered and the depth of each encounter, which provides a “route map” for each MER track. The neurologist or neurophysiologist stands alongside the patient, not in the sterile field (Figure 3.3). In most cases, both the neurosurgeon and neurologist/neurophysiologist are wearing headphones. The brain’s electrical activity is amplified from the deep recording electrode and fed through the headphones and displayed on an oscilloscope. The patient is awake and needs to be able to cooperate with the neurologist and neurosurgeon.
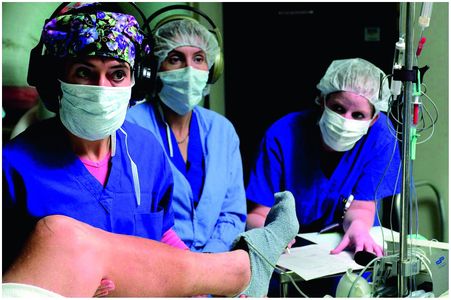
Intraoperative MER. The neurologist or neurophysiologist listens to and watches on an oscilloscope the response of neurons as he/she rapidly and repetitively moves each joint about its axis on the contralateral side of the body. A scribe notes all the responses.
Mapping the sensorimotor region
The first microelectrode track follows the initial trajectory determined by the stereotactic planning. Some centers use a cylinder with five microelectrodes in a concentric array, from which multiple recordings are made during one pass. Other centers use multipass MER, using a single microelectrode, where subsequent tracks are made depending on the electrophysiological findings obtained during the previous track. Single neuronal unit or multi-unit recordings may be obtained. Each unit or group of units is tested for responses to both contralateral arm and leg passive and/or active movement. Within the upper extremity, responses are tested for movement about the metacarpopharyngeal, wrist, elbow, and shoulder joints. Within the lower extremity, responses are tested for movement about the ankle, knee, and hip joints. A movement is made about each joint in the flexion and extension directions separately, rather than a smooth “sinusoidal” motion. Figure 3.4 shows the increase in firing rate of a neuron that is clearly responding to sensorimotor input, rapid wrist extension movement.
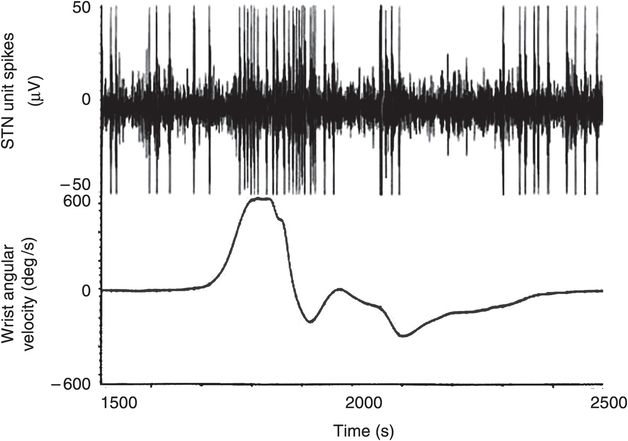
Microelectrode recording (MER) consists of recording the response of neurons to passive and active movement. An STN unit has an irregular, baseline firing pattern and shows an increase in firing rate as the wrist is passively extended. Angular velocity was measured using a solid-state gyroscope attached to the dorsum of the hand.
Boundary and sensorimotor mapping using MER
Microelectrode recording determines accurately the dorsal and ventral borders of the target nuclei and the sensorimotor regions within. Each nucleus trajectory has a distinctive physiological “signature” and will be described briefly separately.
1. STN: the MER track usually passes through the thalamus before entering STN (Figure 3.5). If the pass is anterior there is little neuronal activity in thalamus as the track passes through the reticular nuclei. The more posterior the pass, the more “active” is the spontaneous activity in the thalamus as the microelectrode passes through the ventrolateral nuclei (Voa, Vop, Vim). In these nuclei the electrode may pick up the distinctive activity of “burster” units with intermittent bursts of action potentials and an otherwise quiet baseline. As the electrode passes through the base of the thalamus there is a clear drop in electrical activity as it enters white matter. This silence lasts until the dorsal border of STN is reached, except for the occasional cell that reflects passage through the zona incerta (Zi) (Figure 3.5). The dorsal border of STN is very clear, as there is a dramatic increase in background spontaneous electrical activity. Neuronal activity is irregular and may be oscillatory or bursting in nature. As soon as a single cell or group of cells is isolated, the neurologist begins to map for sensorimotor responses as detailed above. Usually the most dense neuronal activity and sensorimotor “driving” are in the dorsal aspects of the track. At the base of STN there is usually a distinct drop in background activity. The ventral border of STN is followed by entry into SNpr, which is quite distinct electrophysiologically, due to the regularity of firing patterns of SNpr neurons. If there is no or a less than 1 mm gap between STN and SNpr, this is a good guide that the MER location is central in the anterior–posterior plane and one indicator of a good position, given that there was sensorimotor driving within that pass.
2. GPi: the microelectrode track passes through striatum, GPe, and then GPi (Figure 3.6, Figure 3.2b). Each nucleus has distinct electrophysiological features. As the microelectrode passes through the striatum, spontaneous unit firing is evident, which is phasic, i.e., it decreases to silence after a few seconds if the microelectrode remains stationary. New units are activated as the electrode advances. As the electrode moves from striatum to GPe there may be a lamina of white matter, which is silent (Figure 3.6). The entrance to GPe is noted by activation of units with a more tonic pattern, i.e., they persist if the electrode remains in that spot. The background activity remains low, so that this can be difficult to distinguish from striatum. Two distinct types of neurons are found in GPe: high-frequency pausing cells (pausers) and low-frequency bursting cells (bursters). The pausers may be difficult to distinguish from striatal units, but as stated above, their firing will persist; with experience these are quite easy to identify. The bursters are very easy to identify, as they fire at low frequencies with bursts of high-frequency spikes. These are signature units for GPe. As the electrode advances from GPe to GPi there is usually almost 1 mm of silence, reflecting passage through another lamina. The entrance to GPi in PD is characteristic for a dramatic increase in background activity with high frequency (70–90 Hz) discharging units. Sensorimotor mapping as detailed above commences with entry to GPi. As the microelectrode exits GPi, recording continues while the neurologist/neurophysiologist shines a light into the patient’s eyes, to activate fibers of the optic tract. If the optic tract lies immediately deep to GPi, this is a good sign of placement.
3. Vim thalamus: the goal is to place the DBS lead in the Vim/Vop nuclei of the ventral thalamus for tremor. In the thalamus the easiest way to determine the best location for the DBS lead with MER is to identify first the sensory nucleus (ventral caudal – Vc), which forms the posterior border of Vim and is easily identified as the cells respond to light tactile input such as brushing of the skin. Units that have deep kinesthetic responses characterize the border of Vc and Vim; they respond (increase their firing rate) to muscle squeezing and/or tendon tapping. The “kinesthetic” units in Vim respond to classic sensorimotor input (moving of the limbs) as seen in STN and GPi. The other electrophysiological firing pattern that helps to guide placement is the oscillatory firing pattern of units in Vop and Vim, which may or may not be firing at similar frequencies to that of the limb tremor. The mediolateral plane determines the appropriate somatotopic location. The ventrolateral thalamus has an “onion-skin” pattern of somatotopy, where from lateral to medial directions are leg, arm, and face representations. If the patient has significant arm and chin tremor, for instance, the DBS lead should be targeted slightly more medial than if the patient has arm and leg tremor. In our center, anatomical targeting aims for the implant track to be in Vim/Vop, with the target base at the base of the thalamus, at the appropriate mediolateral coordinate.
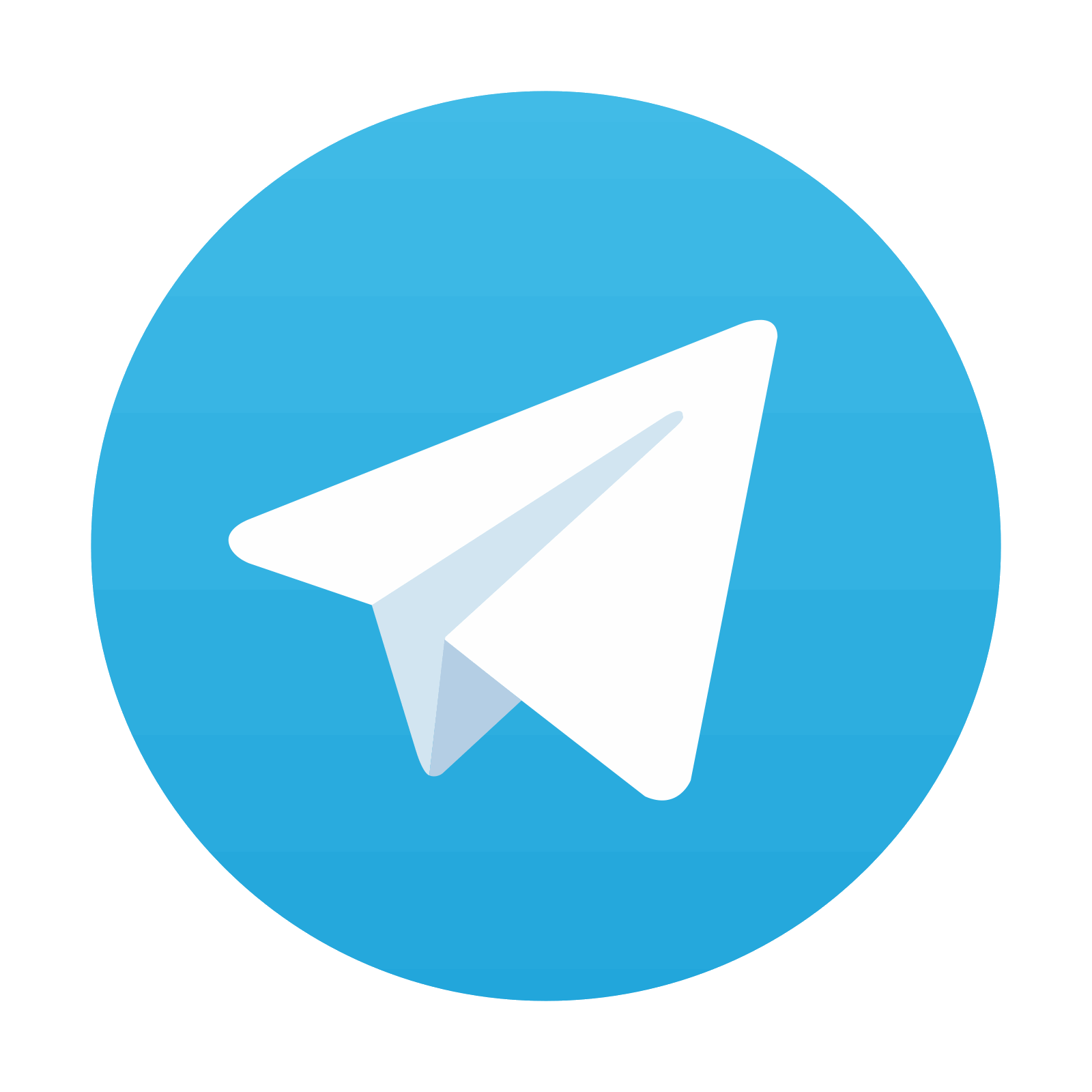
Stay updated, free articles. Join our Telegram channel
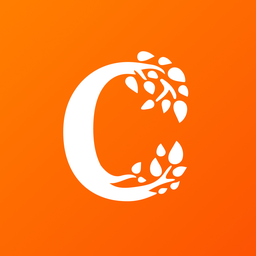
Full access? Get Clinical Tree
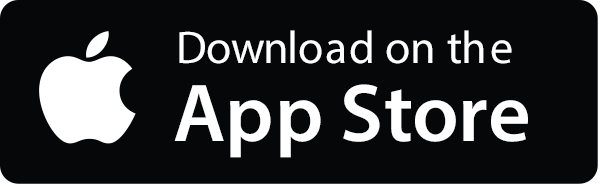
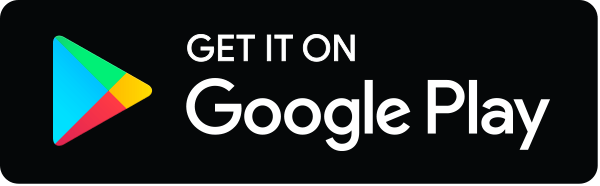