Chapter 1 Techniques in Neuroimaging
PLAIN FILMS
The limited role of plain-film radiography (Box 1-1) in neuroradiology warrants a brief discussion of the technique. Suffice it to say that with plain-film radiography the x-ray beam serves as the source of photon energy and the recipient (film or digital receiver) is the “detector.” The x-ray beam is generated when electrons produced in the cathode of an x-ray tube hit the anode (usually tungsten alloy) target. The electron current is measured in milliamperes (mA), and the potential difference across the x-ray tube is the peak kilovoltage (kVp). Increasing the kVp increases the energy of the electrons flowing toward the anode and therefore increases the amount and energy of x-rays produced. The time that the x-ray tube is in operation is multiplied by the mA to calculate the mAs (milliampere-seconds). Lowering the kVp increases image contrast, but penetration of the photon beam decreases. Increasing the mAs yields greater exposure at the cost of higher current and heat load on the x-ray tube. This has not changed since Roentgen.
Contrast in plain-film radiography is based on the differential attenuation of the x-ray beam by various tissues. As the density, atomic number, and electrons per gram of a tissue increase, the degree of attenuation of an x-ray beam increases. The greater the attenuation of the photons of an x-ray beam, the lighter the image on the film. Thus, metal and bone have a greater degree of attenuation of the x-ray beam than air or soft tissue. Metal and bone will look white on an x-ray film; air is black. By virtue of lower density, fat also has a lesser degree of x-ray attenuation (Fig. 1-1).
COMPUTED TOMOGRAPHY
Parameters and Units
The scale for CT absorption generally ranges from +1,000 to −1,000, with 0 allocated to water and −1,000 to air (Table 1-1). The units are termed Hounsfield units (HU), named to honor the discoverer of the technique. White matter and gray matter are in the 30 to 50 HU range. Hematomas tend to range from 50 to 80 HU, and calcification is generally 150 HU or greater. These values vary by approximately 10 to 25 HU, according to the particular CT machine that is used. Dense bone and metal are the materials at the highest HU range. High protein concentrations (clotted blood, tenacious sinus secretions, the lens of the eye) equate with higher HU values. At values less than 0 one finds the structures that show less CT attenuation than water. Fat is usually in the −40 to −100 HU range. In neuroradiology, the structures with less CT attenuation than fat are relatively limited to air-containing materials (airway, mastoid air cells, sinuses) (Fig. 1-1B).
Table 1-1 HU of Central Nervous System Structures on CT
Structure | HU |
---|---|
Acute blood | 56 to 76 |
Air | −1,000 |
Bone | 1,000 |
Calcification | 140 to 200 |
Cerebrospinal fluid | 0 |
Fat | −30 to −100 |
Gray matter (caudate head) | 32 to 41 |
White matter (centrum semiovale) | 23 to 34 |
CT, computed tomography; HU, Hounsfield units.
CT Perfusion
Iodine-based CT perfusion has also been introduced recently. A large rapid bolus of contrast is infused during continuous rapid scanning of a single slice, and the wash-in and wash-out of the bolus can be analyzed by a computer to generate semi-quantitative images of brain perfusion. Commonly measured parameters are mean transit time (MTT), cerebral blood volume (CBV), and CBF (CBF = CBV/MTT). On CT perfusion images, the differential density between normal brain and hypoperfused brain can be accentuated through computer manipulation to demonstrate areas of ischemia in the brain. The main disadvantages to this technique are the large-bore catheter required (14 to 16 gauge), the rapid injection rate (5 to 10 mL/sec), patient discomfort (one should use nonionic contrast to reduce the “barf” factor), the limited number of slice acquisitions that limit the region of study, and the reluctance of clinicians to give iodinated contrast to patients with strokes. To that end, MR still has an advantage in that only milliosmoles are delivered with gadolinium injections (see discussion of Magnetic Resonance Imaging in this chapter).
Current Role of CT in Neuroimaging
What is the role of CT in neuroimaging (Box 1-2)? It remains the quickest, most efficient screening technique in patients with head trauma. It is the most sensitive imaging study for the detection of subarachnoid hemorrhage (SAH), and is the study of choice for initial evaluation of patients with signs and symptoms suggestive of SAH. CT angiography has gained ascendancy as the mode for screening for aneurysms in patients with SAH in the ED. In a similar vein (equally large), CT perfusion has become an emergency department technique for the evaluation of possible stroke patients and for guiding thrombolytic therapy.
MAGNETIC RESONANCE IMAGING
Relaxation Times
Because T1 and T2 relaxation mechanisms are independent of each other by and large, one can completely lose signal in the x-y axis without having completely returned all the magnetization to the z axis. The T2 or transverse (spin-spin) relaxation is due to dephasing caused by the adjacent hydrogen nuclei, which are not totally in concert with each other. T2 is defined as the time for 63% of the transverse magnetization signal to be lost owing to this natural dephasing process. By and large, T2 values in the CNS are shorter than T1 values. The T1 and T2 values of some normal tissues seen in the CNS are listed in Table 1-2.
Table 1-2 Representative T1 and T2 Relaxation Times of CNS Structures at 1.5 T
Structure | T1 (msec) | T2 (msec) |
---|---|---|
Gray matter | 980–1040 | 64–71 |
White matter | 740–770 | 64–70 |
CSF | >2,000 | >300 |
Muscle (at 1.0 T) | 600 | 40 |
Fat (at 1.0 T) | 180 | 90 |
CNS, central nervous system; CSF, cerebrospinal fluid.
From Berger RK, Rimm AA, Rischer ME, et al: T1 and T2 measurements on a 1.5-T commercial MR image. Radiology 171:273–279, 1989. Data for 1.0 T from Bushong SC: Magnetic resonance imaging; physical and biological principles, St. Louis, Mosby, 1988.
Pulse Sequences
Conventional Spin-Echo Imaging
By varying the repetition time (TR), which is the time between 90-degree pulses, and the echo time (TE), one can obtain T1WI and T2WI. In general, a short-TR (<1,000 msec), short-TE (<45 msec) scan is T1-weighted. A long-TR (>2,000 msec), short-TE (<45 msec) scan is weighted toward proton density. A long-TR (>2,000 msec), long-TE (>60 msec) scan is weighted toward T2 information. The terms short and long here are relative. The intensity of a voxel in a spin-echo sequence is determined by both tissue-intrinsic and scanner-extrinsic factors (Boxes 1-3 and 1-4).
Gradient Echo Imaging
In gradient echo scanning, the magnetization vector of the protons also is tipped off the z axis to the x-y coordinate system (usually less than 90 degrees). As opposed to the 180-degree spin-echo pulse, a rephasing gradient pulse follows the initial flip-angle magnetization. Therefore, the gradient echo scans can be devised to be more susceptible to magnetic field inhomogeneities because of the lack of 180-degree rephasing pulse (Table 1-3). Blood products, iron, calcium, and manganese deposition are seen more readily with gradient echo scanning. These scans are part of routine trauma or stroke protocols searching for blood or in cases where calcified lesions are suspected. Remember, however, that CT is the study of choice for the detection of calcification. When gradient echoes are applied, the most important factors to create T1 or T2 weighting are the value of the angle of nutation, or flip angle, the TR, and the TE. At low flip angles, more T2 weighting is achieved. The lower the flip angle (5 to 10 degrees) and the longer the TE (>40 msec), the greater the susceptibility sensitivity. The cost of extending the TEs to achieve higher susceptibility sensitivity is a reduction in slices available and in signal. To get through the brain, longer scan times are needed. However, gradient echo imaging generally is more rapid than conventional spin-echo imaging and also allows one to obtain bright blood from flow-related enhancement, which is used for MRA and MRV. Three-dimensional gradient echo scanning is also possible and allows very thin slices while maintaining high SNR. The three-dimensional data set may be manipulated into multiplanar reconstructions with relative ease.
Table 1-3 Utility of Gradient Echo Scanning
Feature | Advantage | Clinical Use |
---|---|---|
Shorter TR | Faster | Uncooperative patient, rapid localizer scans |
Can be used without180-degree pulse | Higher susceptibility sensitivity | Better for looking for blood products, calcification |
Can be used with180-degree pulse | “Echoplanar scanning” | Fast imaging, functional studies |
Flow-related enhancement | Bright blood | Basis of time-of-flight MRA, CSF flow imaging |
Less gradient stress | Thinner slices | Cervical spine, sella, temporal bone, MRA, 3DFT images |
Shorter TE | More slices | Thin section T1WI-SPGR |
3DFT, three-dimensional Fourier transformation; CSF, cerebrospinal fluid; MRA, magnetic resonance angiography; SPGR, spoiled gradient recalled; T1WI, T1-weighted image; TE, time to echo; TR, repetition time.
< div class='tao-gold-member'>
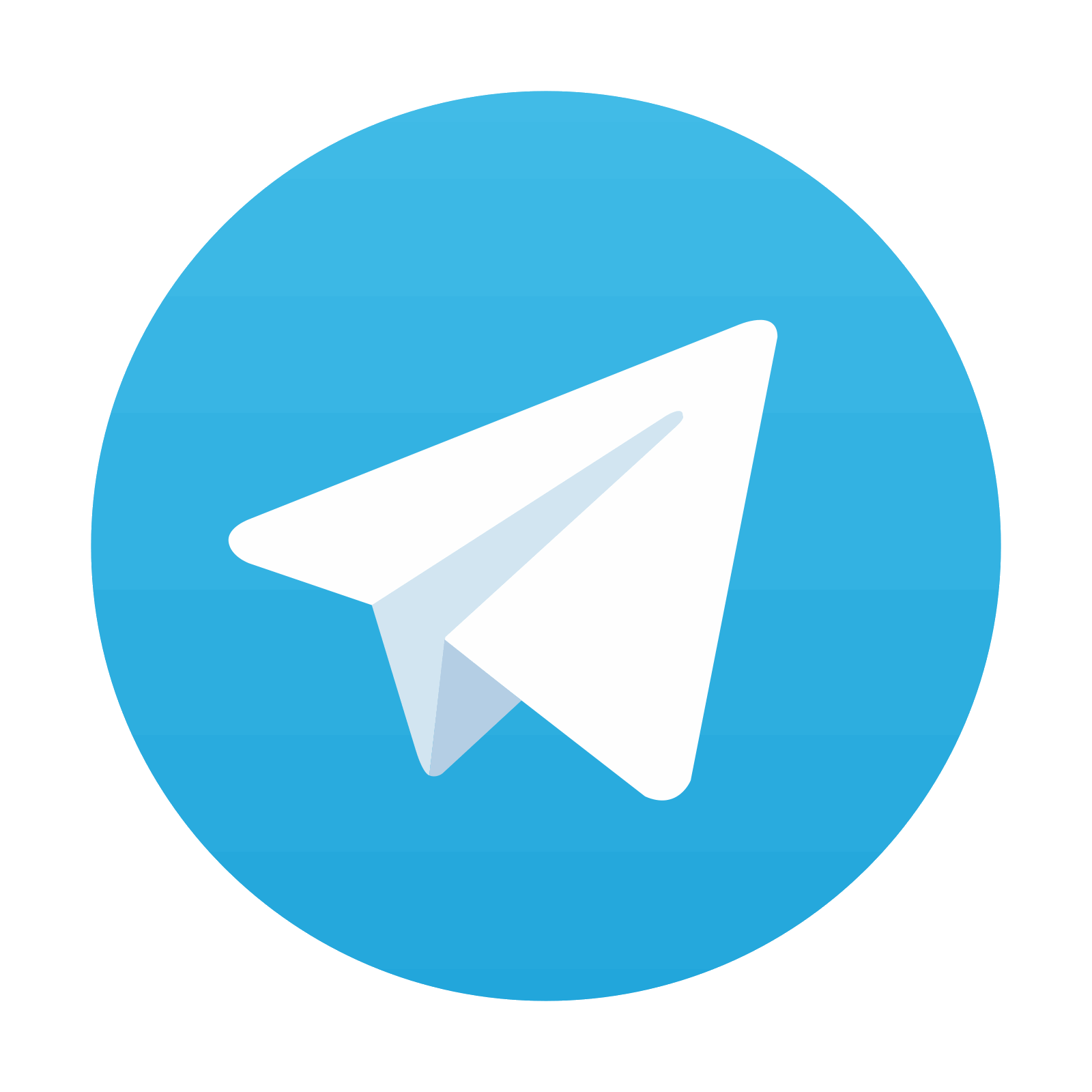
Stay updated, free articles. Join our Telegram channel
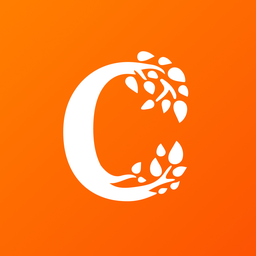
Full access? Get Clinical Tree
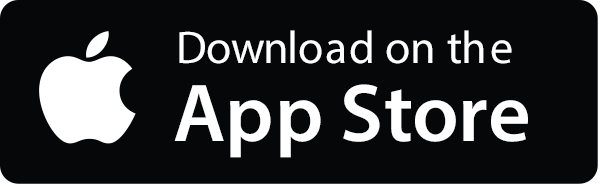
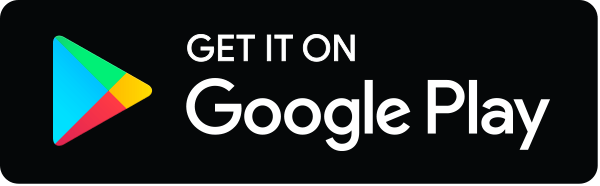