Fig. 1
Hemiparetic gait
TBI patients usually walk slightly faster than patients who had a stroke, with an increased step length. Compared to stroke in patients with TBI, the stance time for the affected limb is diminished, even though it remains increased for the unaffected limb [103]. Lesions affecting the frontal lobes can result in apraxic gait with a rigid pattern and the feeling of being stuck to the ground. This kind of gait is characteristically hypokinetic with slow-speed gait and limitation in limb advancement.
In summary, both patients with stroke and TBI can show a spastic hemiparetic gait pattern. Abnormal muscle activation pattern could lead to problems in motor coordination. In many cases, the cause of the deficits maybe related to spasticity but frequently this is accompanied by muscle weakness with decreased ability to recruit motor units, muscle atrophy, loss of muscle contractile properties, and agonist–antagonist cocontraction. All these factors may adversely impact the generation of functional movements during gait [102].
3 Technology in Rehabilitation
3.1 Biofeedback in Neuroplasticity and Brain–Computer Interface (BCI)
After stroke, 80 % of patients experience acute paresis of the upper extremity and only approximately one-third achieve full functional recovery [9]. Passive phenomena like reperfusion of the penumbra and resolution of brain oedema do account for some of the motor recovery post stroke or in traumatic brain injury. Nevertheless, recent discoveries that neurogenesis and neural reorganization can in fact occur in the adult after CNS injury have been revolutionary for the field of neurorehabilitation and contradict the dogma expressed by Santiago Ramon y Cajal back in the late nineteenth century. Evidence of such neuroplastic changes have been reproduced in both animal [3] and human models [150]. Thence, research on how these neuroplastic phenomena can be exploited for motor recovery is currently a key focus, with neurochemical [11, 20, 134] and neurophysiological [86, 139] evidence of recovery-related neuroplasticity supporting the direction of this work.
A fundamental element in any process of learning is the presence of feedback. From a child who is learning to walk, to a gymnast who is training for a somersault, a real-time stream of multisensory data is continuously flowing to the brain during motor tasks. The integration of data from proprioceptive, visual, auditory, and sometimes even pain receptors helps the brain get a complete picture of the body’s position, motion, and end result of any motor intention. Relaying of such data to the cerebellum, prefrontal, and motor cortices, over a repeated number of successful and failed attempts, forms the basis of motor skill learning [59, 123].
Following stroke or traumatic brain injuries, this motor execution
feedback loop is often disrupted. Damage to motor pathways, either at a cortical or subcortical level, leads to difficulty or complete inability to execute motor intention, which thus breaks the feedback loop very early on. The core of most neurorehabilitation strategies is the aim of reclosure of this loop, either by aiding movement completion or by giving some form of feedback to the central nervous system that is coupled with the initial motor intention.

If one looks at even the most basic of physiotherapy strategies like passive arm movement, the assisted physiotherapist-guided motion is allowing for proprioceptive sensation and visual feedback to reach the brain. A more affective approach—and currently a gold standard in the physiotherapy field—is the use of repetitive, task-specific training for relearning of motor skills needed for activities of daily living [118, 140]. This directly addresses one of the goals of neurorehabilitation as proposed in the latest clinical guidelines, which is to empower patients and families by helping motor function improvement and achievement of the highest level of independence in activities of daily living [99].
3.1.1 Electromyographic (EMG) Biofeedback
With this current knowledge on neuroplasticity in mind, one can start to appreciate the rationale behind the more recent technology-assisted strategies in the field of neurorehabilitation. One of the earliest technologies developed for biofeedback in the field of neurorehabilitation is EMG biofeedback. The mechanism behind this approach is the detection of attenuated electrical activity which occurs in paretic muscles upon motor intention and the conversion of this ineffective data to visual or auditory feedback to the patient—thus reclosing the loop. Research on such setups go as far back as the 1960s and within merely a decade it had become a standardized rehabilitation tool used commonly by physiotherapists [41, 155]. A recent Cochrane review exploring the efficacy and benefits of EMG biofeedback in motor recovery post stroke has identified a number of trials publishing evidence of benefit for motor power, function, and gait recovery when this technology was added on to the standard physiotherapy regime but this has yet to reach statistical significance in view of the limited size of trials and robustness of results [157].
3.1.2 Brain–Computer Interface
Recent advances in neurophysiological signal acquisition and processing techniques have opened the way to a higher form of neurorehabilitative feedback approach, which can completely bypass the peripheral physiological outputs of the body—brain–computer interface (BCI) [13]. Neurophysiological acquisition methods may range from noninvasive technologies like electroencephalography (EEG), magnetoencephalography (MEG), functional near-infrared spectroscopy (fNIR), and functional magnetic resonance imaging (fMRI) to invasive electrocorticography (ECoG). EEG technology still holds several limitations with respect to spatial resolution and quality of brain signal pick-up. Nevertheless, its safety, noninvasiveness, and excellent time resolution makes it one of the most popular signal acquisition media for BCI. Among the earliest accomplishments in the world of BCI was Birbaumer et al.’s success in enabling two ‘locked-in patients’ to communicate using a BCI speller [12]. One of these patients was reported to have eventually learnt to use the BCI on his own to write letters and communicate with his friend [98].
Brain–computer interface systems take advantage of a number of different neurophysiological changes and patterns that have been noted to be associated with particular mental states and tasks [156]. Some of these modalities are dependent on the subject’s intention and can be modulated actively through training. These include the P300 response (a positive EEG deflection 300 ms after a target stimulus is presented to the subject) and the slow cortical potential (SCP) (changes in amplitude that correlate with level of cortical activation). Both of these paradigms have been employed most notably for BCI spellers. Other BCI paradigms are based on passive, natural responses to present stimulus and need no training, like the steady-state visual evoked potentials (SSVEP) (change in the frequency of occipital cortex oscillations that matches the frequency of flashing light presented). These three modalities of BCI are mostly used for assistive purposes, substituting a function that was lost due to motor or speech impairment following conditions like stroke, amyotrophic lateral sclerosis, or traumatic brain injury (Fig. 2).
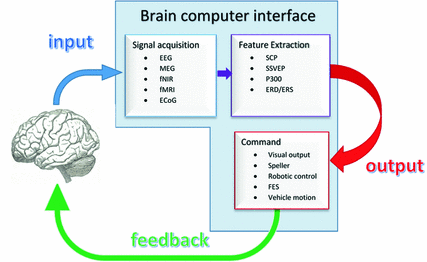
Fig. 2
Brain–computer interface system and the feedback loop
The fourth major modality of BCI is based on motor imagery related changes in oscillatory activity like event related (de)synchronization (ERD/ERS). Desynchronization of EEG activity can be observed following both motor imagery and motor execution initiation, over the motor cortex, in the Mu and beta frequencies, followed by re-synchronization shortly after motor activity. This has become the modality of choice for BCI-based motor rehabilitation i.e., for restorative rather than assistive purposes [131]. Motor imagery (MI) itself has been shown to be associated with motor cortex activation [107, 128]. More importantly, repetitive MI tasks coupled with conventional (physiotherapy and occupational therapy) rehabilitation has been shown to have an added benefit in motor recovery [161]. This is of particular interest when dealing with patients with negligible residual muscle function, in whom standard task-specific or constraint-induced therapy would not be possible.
Initial BCI studies in stroke and traumatic brain injury patients focused on control of mu rhythm via either motor imagery or motor execution in order to either move cursors on screen or move hand orthoses [16]. These assistive approaches were subsequently followed by restorative BCI systems, with or without the use of haptic devices [4] or functional electrical stimulation [29] for proprioceptive feedback. Two examples of BCI-based therapeutic setup with purely visual feedback will be briefly introduced next, while further technological devices will be discussed in subsequent sections.
The first is a computer game-based neurofeedback system developed by Prasad et al. [115] for the rehabilitation of persons with chronic hemiplegic after stroke. The setup consisted of EEG signals acquired from C3 and C4 electrodes (overlying the motor cortex) which are processed online to translate into left and right movements of a ball on a computer screen. Subjects were required to maneuver the ball into a basket by imagining left- or right-hand movement according to the direction required (Fig. 3). Performance throughout the sessions was based both on accuracy classification during the MI task and periodic motor function recovery scores as measured by action research arm test and grip strength. After a 6-week period, improvement in at least one modality of function was noted in all patients and every patient managed to operate the BCI successful with an average accuracy of 60–75 %, suggesting feasibility of such a setup in the context of post-stroke rehabilitation. [115].
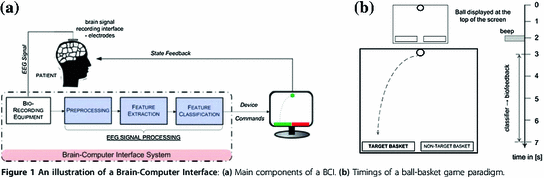
Fig. 3
A computer game-based neurofeedback system driven by EEG-based brain–computer interface—Reproduced with permission from Prasad et al. [115].
In a more recent publication by Cincotti et al. (2012), an elegantly designed BCI-based upper limb rehabilitation system for patients post-stroke is presented. The system is composed of a 32-channel EEG input, driving a BCI software that delivers two concurrent outputs when the patient’s EEG signal is compatible with hand opening/closing motor imagery. The first output is intended for the assisting physician and is in the form of a moving cursor, representing the patient’s mental activity. The second output is a visual feedback intended for the patient and is in the form of realistic images of moving hands, projected onto a white sheet that is placed over both of the patient’s hands (Fig. 4). In this way, attempts at hand movement lead to the visual illusion of the paretic hand moving, as projected onto the screen superimposed on the paralyzed hand. This system was installed in a rehabilitation ward and tested on 29 stroke patients in whom increased alpha and beta reactivity was noted post training, along with moderate increases in functional outcome measures when compared to control subjects testing non-BCI MI practice.
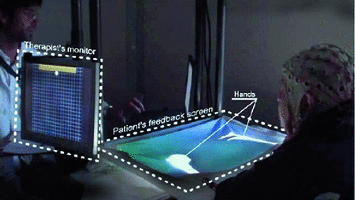
Fig. 4
EEG-based BCI upper limb rehabilitation system, giving both motor imagery information to the therapist and visual realistic hand motion feedback to the patient—Reproduced with permission from Morone et al. [94]
3.1.3 Conclusion
As its name implies, brain–computer interface is merely a new interface that can translate a person’s intentions into numerous outputs of various forms. Thus, the present challenge in neurorehabilitation research is the development of useful BCI applications and platforms that can offer more effective and efficient motor recovery to patients suffering from stroke and traumatic brain injury.
To date, most studies explore the benefits of MI-BCI rehabilitation in conjunction with standard physiotherapy, and so robust statistical evidence of superiority of MI-BCI-driven rehabilitation over standard active motor training is still difficult to prove and further randomized trials need to take place. Confounding factors to the observed beneficial effects of MI-BCI rehabilitation—albeit desirable themselves—include the increased willingness and time spent by patients doing active motor training within the BCI setup itself when compared to those in the standard, repetitive task-training groups.
The potentials for BCI in this context are various: visual, proprioceptive or, any other output that is coupled with motor imagery/intention can reinforce biofeedback to the patient and provide valuable information to the clinician regarding the patient’s engagement and performance in these previously invisible MI tasks. Finally, innovative outputs and applications driven by MI-BCI can increase patient’s motivation and engagement in his rehabilitation process, through the use of media like video games, engaging work-out sessions, or simply through the confidence-boosting sight of his arm actually moving on command, made possible only through BCI-driven robotics or functional electrical stimulation.
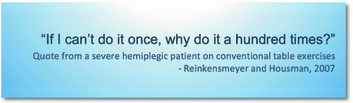
3.2 Virtual Reality-Based Rehabilitation
Rehabilitation technologies such as virtual reality are based on motor learning principles and could be implemented in order to compensate, restore, and recover cognitive impairment and loss of sensorimotor function caused by stroke and TBI.
Virtual reality can be defined as an approach to user–computer interface that involves real-time simulation of an environment, scenario, or activity that allows for user interaction via multiple sensory channels [17].
The rationale for using virtual reality training on brain injury rehabilitation is because during therapy a repetitive massed practice of relevant functional tasks based on imitation and movement observation could be useful to facilitate targeted brain networks and potential neuroplastic changes. Functional and motor recovery observed during virtual reality task-oriented therapy may be linked to induced neuroplasticity changes with a mirror-neuron system activation, reorganization of damaged motor cortex, decreased aberrant cortical hyperexcitability on unaffected hemisphere, and at synaptic level synthesis of neurotrophic factors (BDNF) that encourage axonal sprouting and dendritic spine formation [15, 109, 159].
Virtual environments are characterized as immersive, where a three-dimensional environment is displayed allowing to change visual perspective with head movements (head-mounted visual displays, virtual caves), semi-immersive with three-dimensional fixed visual perspective presentations, or nonimmersive in the case of two-dimensional presentations displayed on a screen with or without interface devices as keyboard, computer mouse, or a joystick. There are some commercially available devices such as the IREX system (from GestureTek, Canada), Nintendo Wii , Sony Playstation EyeToy, Microsoft Kinect (Fig. 5). Furthermore, there has been virtual reality systems designed to be coupled to haptic and robotic devices to provide interaction forces and sensorimotor feedback between the user and the virtual environment. Examples of combined robotic and haptic systems include the Rutgers Ankle Rehabilitation system, Cybergrasp, CyberGlove, Gentle-S, PneuWrex , Mit-Manus, and Armeo Power. They all have been used with the purpose of increasing the feeling of immersion and user interaction into the virtual scenario. Haptic devices add precision to tracking motion, providing a powerful multisensory feedback that contributes to improve real-world activity level motor outcomes [19].
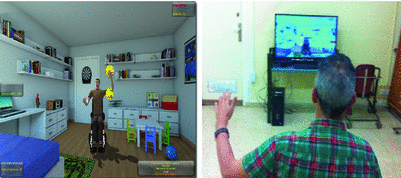
Fig. 5
A kinect-based virtual reality system. With permission of Complejo hospitalario de Toledo- Spain
Feedback is crucial for user’s engagement, motivation, and concentration during virtual therapy and has been determined essential to increase effect . In addition to visual and auditory feedback, tactile and force feedback provided by haptic devices increases the user interaction with the virtual environment and objects. Augmented feedback also could be implemented during sessions using knowledge of results (KR) or knowledge of performance (KP- desirable/ undesirable movement patterns).
Usually, virtual reality therapies are applied to patients with low or moderate spasticity (Ashworth scale score equal or less than 2), and mild or moderate upper limb paresis. The presence of certain degree of active movements and manipulation ability (active wrist extension above 20
, metacarpophalangeal finger extension above 10
, and approximately between 30 and 42 points in upper extremity Fugl-Meyer assessment) seem to be necessary for participation in virtual therapy programs. Hand dexterity scores lower than 45 in Box and block test could be also considered a criterion for therapy admission.


Treatment sessions can vary widely in terms of number and duration between different studies. Duration of 40 min to 1 h, delivered 3–5 days a week along a three–four week period, is common [83].
Usually, patients with both types of stroke, ischaemic, or hemorrhagic are included in virtual reality therapy. A recent study has shown, in line with previous data with more classical interventions [104] a differential response depending on etiology of stroke. Although all participants achieved motor improvements, patients affected by hemorrhagic stroke improved significantly more on FIM scores and kinematic parameters compared to ischaemic stroke patients [64].
Majority of patients are treated predominantly during a subacute or chronic phase (greater than 3–6 months) after brain injury, because in many cases acute phase deliver of this therapy results in impractical due to an unstable medical condition or severe plegia.
Virtual reality therapy is typically applied to patients without cognitive impairments, but many times stroke and TBI patients show cognitive and perceptual disorders. The minimal cognitive and perceptual requirements to use virtual rehabilitation in an effective manner still remain unidentified.
3.2.1 Virtual Reality for Upper Limb Rehabilitation in Stroke and TBI
An increased number of studies have been proposed in the last decade to evaluate the scientific evidence for the effectiveness of virtual reality in upper limb rehabilitation after TBI or stroke.
Regarding virtual reality effectiveness for upper limb motor recovery in stroke patients, a moderate beneficial effect has been reported. In a systematic review by Henderson et al., the authors found that compared to conventional therapy, there is a moderate level of evidence (2b) on the effectiveness of using nonimmersive virtual reality training for upper limb rehabilitation [50].
In a meta analysis that reviewed five randomized controlled trials about virtual reality therapy for subacute and chronic stroke patients (daily sessions, 5 days a week, during 4–6 weeks), authors found improvements on upper limb motor function, with increasing Fugl-Meyer scale scores between 13.7–20 %, at the end of treatment when compared to controls (3.8–12.2 %) treated with conventional occupational therapy [124].
A recent Cochrane review [78] analyzed 19 studies with 565 participants. In three of them (101 participants), they achieved significant statistically differences regarding ADLs performance in patients with virtual reality therapy (standardized mean difference 0.81; IC 95 % [ 0.39–1.22]). However, authors concluded that there was insufficient evidence about the effectiveness of virtual reality therapies to significantly increase grip strength.
A recent review of current state of post-stroke virtual rehabilitation [42] analyzed eight studies of upper limb and concluded that virtual reality could induce a moderate improvement on upper limb motor function with a small additive effect in terms of real-world activity daily living motor outcomes in subjects performing intervention with haptic feedback.
Another recent study [79] reviewed ten studies examining effectiveness of virtual reality-based rehabilitation regarding upper limb and hand fine motor skills in chronic stroke patients. Significant improvements were found in finger fractionation, finger tracking, and time from peak hand velocity at the moment an object was lifted from a table. Moreover, in five of the ten studies of this review, significant improvements were reported regarding transfer of virtual reality gains to real-world tasks when assessed by the Jebsen Taylor hand function test.
In a recently published systematic review and metaanalysis, twenty six upper and lower limb controlled trials compared virtual reality to conventional therapy in stroke patients [82], demonstrating a moderate effect in favor of virtual reality therapy. Time post-stroke and type of virtual therapy intervention (commercial gaming, semi-immersive, immersive virtual environments) did not significantly affect the outcomes. In addition, virtual reality therapy induced significant improvements across domains of International Classification of Function, Disability, and Health. (ICF- World Health Organization. Geneva, 2001). For body function level, there was a significant benefit of virtual reality therapies compared to conventional therapy, with an overall effect size G = 0.48, 95 % CI [0.27,0.70]. For activity level outcomes, they found the same benefit with an overall effect size G = 0.58, 95 % CI [0.32, 0.85], whereas for participation level outcomes, the overall effect size was G = 0.56, 95 % CI [0.02,1.10]. However, authors accept that results should be interpreted with caution due to considerable variability within interventions.
The evidence in favor of using virtual reality for upper limb rehabilitation in TBI patients to improve motor function still is very limited.
In a four-patient study, [52] used a virtual reality system consisting of a computer, software, and a motion-tracking device. Prerecorded arm movements of a virtual “teacher” and the patient arm movements using a motion-tracking capture system were displayed on a computer screen. The patient was asked to mimic the virtual teacher movements, and the difference between movement trajectories provided augmented feedback. In this study, three out of the four patients improved upper limb function, and movement trajectories were smoother, straighter, and more accurate after the therapy. Moreover, patients achieved higher Fugl-Meyer scale scores and were able to generalize learned skills to real-world performance.
Virtual reality systems for TBI patients seem to improve accuracy of movement for both hands (left p = 0.01; right p = 0.02), speed and efficiency for right hand (p = 0.01 and p = 0.002, respectively), and bilateral hand dexterity assessed by Box and Block Test [95].
Appropriate arm-postural coordination is crucial to perform reach and grasp activities without abnormal compensatory strategies (trunk displacement) and to carry out activities of daily living in standing position (bathing, dressing, cooking).
A study of a virtual reality system for arm-postural coordination using the World Viz Vizard software (Santa Barbara CA), integrated with a six-camera system for motion capture and a custom-made 3D- immersive videogame. Patients use large arm movements to control their avatar trying to reach the maximum number of targets. Participants with mild-to-moderate TBI using this virtual system improved arm movement time, arm postural coordination and movement trajectory, forward reach, and single-leg standing [143]. There was no follow-up to assess retention and duration of improvements.
3.2.2 Virtual Reality for Lower Limb Rehabilitation in Stroke and TBI
Efficacy of virtual reality technology to improve walking and balance for post-stroke and TBI patients has been studied for its clinical application during the last ten years. Hardware and software of virtual reality systems created for this purpose ranged from fully immersive systems combining motion platforms, instrumented treadmills, motion capture systems, and surround sound systems (i.e., Computer-Assisted Rehabilitation Environment-CAREN) to nonimmersive gaming-based commercially available systems (i.e., Nintendo Wii), and virtual reality systems coupled to haptic or robotic devices (i.e., Rutgers Ankle Rehabilitation System).
Improvements on balance and ambulation could be due to task-specific training and the virtual reality elements that simulate real-world environments and motivate patients to practice.
For stroke patients, most studies have been carried out during the chronic phase post stroke. In this population, combining virtual gait training with conventional therapies seems to be more effective than application of virtual training alone.
Some studies show that chronic stroke patients who received additional 30 min per session of virtual reality walking therapy added to conventional physical therapy achieved significant improvements on balance, gait velocity, cadence, and step length, compared to controls without virtual therapy [24, 63]. Therefore, even a short time of virtual training a day could yield significant gait improvements.
A differential effect in terms of biomechanics and functional outcomes has been found between nonimmersive and immersive haptic–robotic virtual systems.
A positive impact on gait biomechanics has been shown when patients are trained with a force-feedback robot interfaced virtual reality system. In a single-blind randomized control study, subjects in the robot interfaced virtual reality group demonstrated significantly larger increase in ankle power generation, ankle range of motion (ROM), and knee ROM during swing phase of gait, compared to controls [93].
In contrast, a randomized controlled trial [44] showed that chronic post-stroke patients that followed balance and gait training with a nonimmersive video-gaming system did not achieved significant improvements on Fugl-Meyer assessment, Berg balance test, time up and go, and 6-min walk test compared to controls that continued with normal activity without receiving any special intervention. However, additional studies are needed to establish real efficacy of nonimmersive commercially available devices for virtual gait training.
Evidence about virtual reality therapy for gait and balance recovery in acute stroke patients is still limited. In a recent randomized controlled trial [91] that studied 59 acute stroke patients, the treatment group (n = 30) received standard rehabilitation plus virtual reality therapy (10–12 sessions, 30 min per sessions, 3 weeks) that included some exercises that challenged balance while standing (soccer, snowboarding). The control group (n = 29) received the same conventional rehabilitation plus identical exposure to virtual therapy without balance challenging exercises (performed sitting). Patients in virtual training balance exercises achieved after therapy greater improvements on 2-min walk test and time up and go test, diminishing lower limb impairment assessed by Chedoke-McMaster Leg domain compared to controls.
Studies with acute TBI patients and virtual reality-based gait training are also scarce, but at least for chronic patients some studies had shown feasibility and efficacy of this therapy, with improvements on gait and balance confidence [138, 141].
For mild-to-moderate chronic TBI patients, arm–leg coordination movements and dynamic postural stability gains have been found using an Xbox Kinect sensor with an interactive customized virtual reality games and scenarios [144].
In addition to this evidence of efficacy, studies point to the feasibility of virtual therapy implementation. Thus, nonimmersive virtual training added to conventional therapy could lead to improvements on walking distance, gait speed, and balance compared to usual therapy, with high therapy compliance and patients attending the majority of sessions without any reports of adverse events [89].
3.2.3 Conclusions
At the present time, studies indicate moderate evidence about the effectiveness of virtual reality technology to improve gait, balance, and upper limb motor function in stroke and TBI patients. Combined interventions adding virtual training to conventional rehabilitation appear to yield better motor and functional outcomes than a single intervention.
There are still some challenges to attain effective translation of learned motor skills in virtual environments to real-world activities of daily living performance. Emerging evidences suggest some progress in this issue, as represented by the positive impact found with virtual therapies across ICF domains.
Nevertheless, outcomes obtained from different studies should be interpreted with caution due to considerable variability within interventions (dosage, intensity, duration), small sample sizes, variable design quality of studies, and lack of detail provided about “conventional therapy.” Finally, reports of cybersickness or adverse side effects (dizziness, headache, pain) after using virtual reality are scarce or minimal. Future studies should be considered to determine whether virtual reality training could affect patients condition, facilitate recovery, or interfere directly or due to adverse effects with functional recovery.
3.3 Robotics and Haptic Devices
Robotics in the medical field requires the convergence of expertise in robotics, medicine, and computer science, and the identification of specific robotic system design demands is in various stages of development.
In recent years, there have been significant developments in the design of robotic system for applications in surgery, rehabilitation, prosthetics, and assistance directed at the elderly or the disabled.
In particular in the field of rehabilitation, robotic systems may have the potential to reduce the demands.
Human operators in order to cope with the increasing growth demand and potential for injury by reducing the staff effort introduce more effective rehabilitation protocols.
Robotic systems are also called upon to address the demands for home care for the elderly, especially in relation to the execution of repetitive tasks.
Robotic technology can provide support to rehabilitation therapy [146]. Early robotics, starting in the late 1950s, focused on large manipulators to replace workers in factories who were performing dirty, dangerous, and undesirable tasks. The rehabilitation robots were based on previous designs in the field of prosthetics, where devices have been developed for the substitution of function of upper and lower limbs, some of which are in widespread use in rehabilitation clinics. This chapter will focus on devices for the rehabilitation of the upper limb and review some of the most used.
3.3.1 Robotics Devices
Robots for upper limb rehabilitation generally consist of robotic arms with several degrees of freedom, the end of which is connected to the hand or arm of the patient. Many research groups have developed robotic devices for upper limb rehabilitation, for example, Massachusetts Institute of Technology (MIT) Manus [69], Assisted Rehabilitation and Measurement (ARM) Guide [116], Mirror Image Motion Enabler (MIME) [18], Bi-Manu-Track [51], GENTLE/S [26], Neurorehabilitation Robot (NeReBot) [120], REHAROB [142], Arm Coordination Training 3-D (ACT
[136], and ARMin [97].

Generally, you can follow on the computer screen a representation of the movements of the robot. The interaction between patient motion and movement of the robot can be done in various ways. The robot can drive limb movements of the subject completely, without any subject active participation; or you may demand a greater participation by the user, who must strive to make the move. The user must prioritize positioning task performance, e.g., move the robotic arm so that its position in space, represented graphically on the computer screen, coincides with a desired position, also shown in the form of a ‘target’ on the screen. The task of the robot, in these scenario, is to encourage parallel movement of the patient, e.g., applying guiding resistance in the event of deviations from the desired trajectory, or to apply assistance if the patient has difficulty initiating or continuing the movement. The utility of these robot systems is shown by numerous clinical studies [114].
3.3.2 Haptics Devices
Haptics is a term that was derived from the Greek verb “haptesthai” meaning “of or relating to the sense of touch.” It refers to manual sensing and manipulation of surrounding objects and environments through the sense of touch. The “touching” of objects and or environment can be made through these devices and the objects and environments can be real, virtual, or a combination of both. Also, the interaction may or may not be accompanied by other sensory modalities such as vision or audition [38].
The training with these devices is based on exercise therapy modalities that the literature and/or clinical practice indicate may help restore upper limb motor control and function [37].
There are two modalities to restore mobility, passive, and active mobilization. In the passive mode, the robotic device moves the patient’s arm. In the active mode, the movement is either partially assisted by the robotic device, or resisted by the robotic device. An example of passive motion intervention is a system that provides bimanual mobilization, where the movement of the unaffected arm is mirrored by simultaneous passive movement of the affected arm provided by the robotic device.
For example, the device Phantom permits simulation of fingertip contact with virtual objects. A pen-like stylus tracks the pitch, roll, and yaw and x, y, and z Cartesian coordinates of the virtual point probe. Its actuators communicate forces back to the user’s fingertips as it detects collisions with virtual 3-D objects, simulating the sense of touch [88]. Other haptics devices are Omega3 [45], Falcon [87], and haptic knob for rehabilitation of hand function [72].
For ambulation rehabilitation, the science behind exercise in persons with neurologic disease supports treadmill training over “conventional therapy [33]. What constitutes “conventional therapy” is highly variable and not well described in the literature. This area only recently has been given more study attention [30]. Traditionally, the conventional physiotherapy approach focuses on strengthening and practicing single selective movements or various neurofacilitation techniques. Conventional therapy methods, however, do not specifically emphasize the activity of ambulation. Limited evidence exists to support the effectiveness of these techniques in the restoration of walking ability [47, 67, 80, 148].
Many rehabilitation paradigms have been developed to promote recovery of lower extremity function and walking through task-specific training. Walking on the treadmill alone [77, 119] or in combination with body weight support (i.e., body weight supported treadmill training [BWSTT]) [27, 149] has become an increasingly popular option in the past several years. Although recent reviews of the literature have not demonstrated different outcomes of BWSTT for patients who have had a stroke [31, 36] based on task-specific approach, gait training is the best way to improve the walking pattern [28]. Such repetition is thought to facilitate the integration of remaining and altered sensorimotor systems in persons with either an acute or chronic brain injury [53, 137].
Positive changes in larger cortical representation have been demonstrated in patients with a TBI [60, 105]. Rehabilitation efforts that promote motor recovery of ambulation activity through task specificity and repetition for persons with a TBI can be complicated by a number of different factors: (1) the potential for multiple motor impairments, both of a pyramidal and extrapyramidal nature; (2) the presence of cognitive or communication impairment; (3) the need for a high number of repetitions as part of the training; and (4) the high level of manual assistance required to help the patient during gait training. These factors combine to make task-specific training for persons who have sustained a TBI very labor intensive, time consuming, and costly. To address some of these limitations, locomotor therapy with a robotic device has been introduced as a task-specific technique to apply precise movement training that may increase the efficiency and/or effectiveness of this type of intervention. Some of the benefits of robotic-assisted treadmill training include more intensive and prolonged training modes, consistent movements, and a reduction in manual labor required from the therapist. Although considerable literature exists on the effectiveness of locomotor training with robotic-driven therapy on spinal cord injury populations [25, 57, 153], surprisingly little published research is available regarding persons with a brain injury. In some studies of persons who had a stroke, investigators found better outcomes with robotic-assisted training compared with conventional training [127].
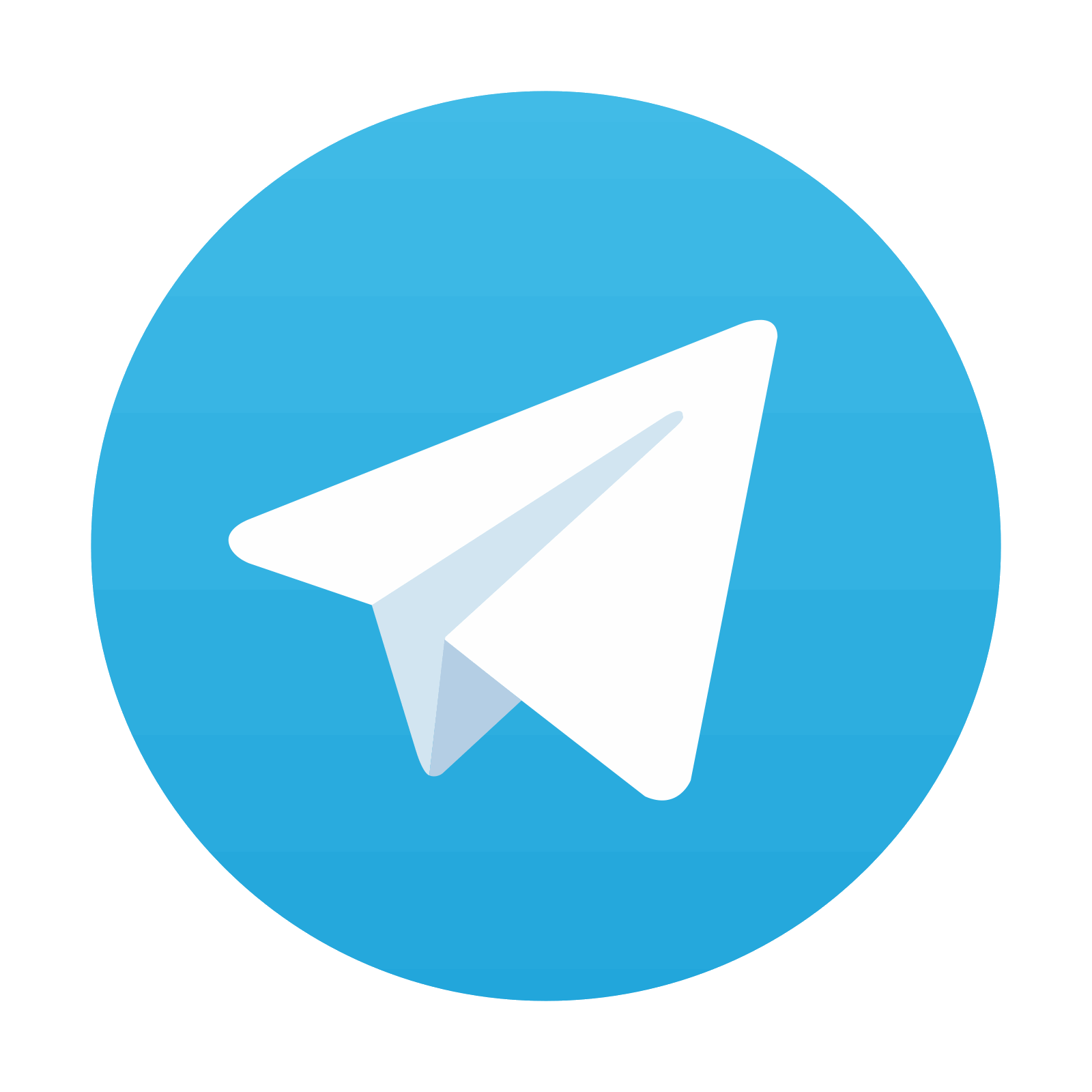
Stay updated, free articles. Join our Telegram channel
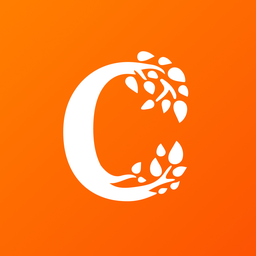
Full access? Get Clinical Tree
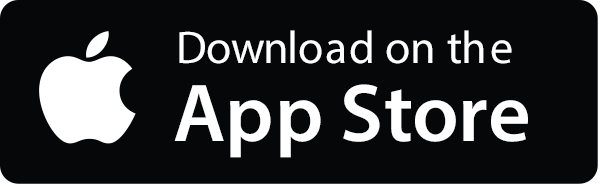
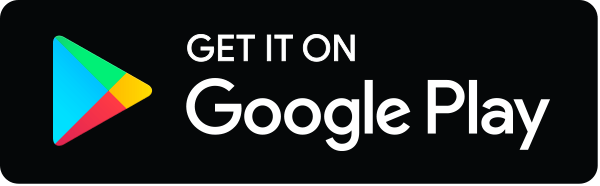