Fig. 1
Spinal cord segments (Modified with permission from Cancer Research UK/Wikimedia Commons)
In complete SCI, there is no function, sensation, and movement below the level of injury; both sides of the body (ipsilateral and contralateral to the lesion) are equally affected. On the other hand, in incomplete SCI, some function is preserved below the level of the injury. A person with an incomplete injury may have asymmetries between both sides of the body in terms of mobility or sensation. Clinically, complete SCI occurs less frequently than incomplete SCI, and therefore, most patients experience some functional recovery after the initial stage of spinal shock and inflammation (acute phase of the injury).
2 Clinical Evaluation of SCI
Individuals with SCI typically present with a gamut of neurological and functional impairments, depending upon the spinal level of the lesion and the extent of the injury. Furthermore, the clinical signs and symptoms also change with time (starting from onset of the SCI) as the acute inflammatory processes subside, and intrinsic recovery starts to occur. Therefore, it becomes very important to document the clinical signs as well as the functional performance in order to monitor patient’s progress and appropriately adjust therapeutic strategies and goals to maximize functional outcomes. Given the physical rehabilitation focus of this chapter, the scales pertinent to assessing sensory and motor function are primarily discussed below, while assessments of the autonomic nervous system function are excluded.
American Spinal Cord Injury Association (ASIA) Scale:
The neurological assessment in SCI is performed based on the guidelines of the ASIA scale [33, 38], which relies on a systematic exam of the motor and sensory functions. The motor evaluation component comprises the testing of 10 muscles: 5 in the upper limbs (UL) and 5 in the lower limbs (LL) wherein muscle strength/function is scored between 0 and 5 according to the guidelines presented in Table 1.
Sensation is also assessed and scored on a scale of 0–2 in which 0 indicates anesthesia; 1, altered sensitivity (including hyperesthesia); and 2, normal sensory function. Table 2 presents the five levels of neurological impairment in SCI (ASIA).
Penn Spasm Frequency Scale (PSFS):
Penn et al. defined a 5-point scale (see Table 3) categorizing the frequency of occurrence of spasms, [27, 44]. Priebe et al. in [46] modified the Penn spasm frequency scale (PSFS), adding a second index to account for the individual’s level of spasticity.
Walking Index for Spinal Cord Injury (WISCI) Scale II:
The WISCI II indicates the ability of the user to walk after a SCI [15]. This scale scores the individual between 0, indicating most severe level of disability, and 20, indicating minimal impairment.
Ashworth and Modified Ashworth Scales of Spasticity:
Spasticity, defined as a velocity-dependent response to passive stretch [30, 35], is one of the most important consequences of central nervous system lesions [7]. The Ashworth spasticity scale was developed as a simple clinical classification for assessing the effects of an antispasticity muscle relaxant drug (Carisoprodol) in multiple scleroses [4]. Ashworth scale (AS) is a 5-point scale based on subjective clinical assessment of patient’s muscle tone [27]. Modified Ashworth scale (MAS) adds an extra value to the scale (1) to include hemiplegic patients [27]. Due to its simplicity, therapists have adopted AS and MAS scales for quantification of spasticity in wide a variety of diseases and conditions, including SCI [23]. MAS scale is shown in detail in Table 4.
Table 1
ASIA motor grading scale
Index | Description |
---|---|
0 | Total paralysis |
1 | Palpable or visible contraction |
2 | Active movement, full range of motion (ROM) with gravity eliminated |
3 | Active movement, full ROM against gravity |
4 | Active movement, full ROM against gravity and moderate resistance in a muscle-specific position |
5 | (Normal) Active movement, full ROM against gravity and full resistance in a muscle-specific position expected from an otherwise unimpaired person |
5* | (Normal) Active movement, full ROM against gravity and sufficient resistance to be considered normal if identified inhibiting factors (i.e., pain, disuse) were not present |
NT | Not testable (i.e., due to immobilization, severe pain such that the patient cannot be graded, amputation of limb, or contracture of >50 % of the range of motion) |
Table 2
ASIA Impairment scale (AIS)
Index | Description |
---|---|
A | Complete sensorimotor injury |
B | Incomplete sensory, complete motor injury |
C | Nonfunctional incomplete sensorimotor injury |
D | Functional incomplete sensorimotor injury |
E | Normal sensorimotor function |
Table 3
Penn spasm frequency scale
Index | Description |
---|---|
0 | No spasms |
1 | Stimulus induced spasms |
2 | Spasms occurring less than once per hour |
3 | Spasms occurring more than once per hour |
4 | Spasms occurring more than ten times per hour |
Table 4
Modified Ashworth scale for spasticity
Grade | Description |
---|---|
0 | No increase in muscle tone |
1 | Slight increase in muscle tone, manifested by a catch and release or by minimal resistance at the end range of motion when the affected parties moved in flexion or extension |
1 ![]() | Slight increase in muscle tone, manifested by a catch, followed by minimal resistance throughout the remainder (less than half) of the range of motion |
2 | More marked increase in muscle tone through most of the ranges of motion, but the affected part is easily moved |
3 | Considerable increase in muscle tone, passive movement is difficult |
4 | Affected part is rigid in flexion or extension |
3 Rehabilitation Techniques for Spinal Cord Injury
Restoration of functional independence in activities of daily living (ADL) is a priority for individuals with SCI, along with bladder and sexual functions, and mitigation of circulatory and respiratory issues, pressure sores, and psychological problems. Therefore, physical rehabilitation continues to remain a mainstay in the treatment of SCI. Some decades ago, SCI meant confinement to a wheelchair and a lifetime of medical co-morbidity [50]. Advances in neuroscience have provided hope for stimulation of spinal cord regeneration and eventually complete functional restoration. Various solutions have been explored for SCI treatment and rehabilitation, each with its own advantages and limitations. Traditionally, rehabilitation has focused on functional restoration by maximizing residual motor skills through therapeutic exercise, or by overcoming losses with teaching of compensation techniques and use of assistive devices in patients.
While the complete repair of injury by regeneration techniques would be ideal, such techniques are still in early development (including stem cell therapies), and will need to be translated from preclinical to clinical therapies (for additional details refer to the section Rehabilitation Techniques for Complete SCI: Neural Regeneration). Nevertheless, for such neural repair to work, neurons within the spinal cord involved in generating rhythmic movements still need to be functioning. Therefore, the physical rehabilitation techniques currently used for functional restoration could be seen as a means of keeping neurons active until regeneration treatments become viable [21].
Clinical approaches for functional restoration are usually based on neuroplasticity to some extent, which consists of facilitating the adaptive reorganization of the sensory-motor systems within spared neuronal circuits to the loss of function due to injury/lesion [14]. There are several potential techniques for rehabilitation of SCI subjects. These approaches can typically be classified based on the severity and level of the injury, as treatment approaches will vary depending on the lesion. According to Table 5 [14], the goal of rehabilitation guides the selection of the most appropriate treatment approach based on the completeness of injury, such that it can maximize functional outcomes.
Severity | Treatment | Goal | Current state |
---|---|---|---|
Complete SCI (AIS A) | Compensation by assistive devices (such as BCI) | ADL independence | Established |
Neural repair | Low level of motor function (hand or locomotor) | Still in translation to human | |
Incomplete SCI (AIS B/C) | Epidural electrical or pharmacological stimulation. | Low level of motor function (hand or locomotor) | Still in translation to human |
FES (Functional Electrical Stimulation) and orthosis | Tenodesis grasp | Established | |
Assisted stepping | Established | ||
ISMS (Intraspinal Microstimulation) | Restore limb function | Still in research | |
Intracortical Microstimulation | Restore sensation | Still in research | |
Incomplete SCI (AIS C/D) | Functional training | Active hand function | Established |
Restricted locomotor function | Established |
3.1 Rehabilitation Techniques for Complete SCI
The treatment approach for complete injury usually is more focused on compensating the functional movements required for activities of daily life (ADL) with assistive devices such as powered wheelchairs, and more recently powered robotic lower limb exoskeletons ([42]. Another issue that is still in development is the neural regeneration aiming to achieve the recovery of the lower level motor functions to some extent so that the patient can self-initiate the movement of an assistive device.
Neural Regeneration
According to [21], while the peripheral nervous system (PNS) has the capacity to regenerate itself, the central nervous system (CNS) has limited capacity of regeneration after a damage occurs. In order to make the correct connections, i) cells have to be alive or be replaced (neural or tissue transplantation), ii) the environment has to be permissive for axonal growth, iii) correct signaling to select the desired target has to be provided, and iv) axonal remyelination has to be allowed. Some studies have demonstrated that application of electrical fields can directly affect the orientation and regeneration of axons [24]. Apart from this, spinal cord prosthetic devices to promote host tissue regeneration, rewiring, and plasticity are also being developed [22]. Passive SC prostheses are usually based on artificial scaffolds impregnated with cytokines to promote the surrounding tissue regeneration. On the other hand, the use of active SC prostheses is also aimed to the treatment of pain, bowel or bladder dysfunction, spasticity management, and motor control of limbs and trunks. Regeneration is considered as the solution for the complete repair and restoration; however, it is still under investigation and some promising results have been recently published [57]. In this Phase 1 clinical study, three human subjects with SCI were transplanted with autologous mucosal olfactory cells in the injured SC segments, and were provided with post-operative neurorehabilitation. Interestingly, neurophysiological examinations showed improvement in spinal cord transmission and activity of lower extremity muscles in surgically treated patients but not in patients receiving only neurorehabilitation. These initial results are encouraging and further investigations with a larger population will elucidate the mechanism of neural recovery after such transplantation, and will help develop more targeted and effective treatment approaches.
Neuroprostheses and Brain–Computer Interfaces
When there is a severe paralysis due to SCI, assistive devices normally help achieving independence in activities such as grasping, driving a wheelchair, or triggering an active orthosis. In this context, neuroprostheses have attracted considerable attention, as they can serve as a potential tool for intuitive user-driven control of an assistive device. This approach is typically useful for providing intuitive control in extreme disability to provide potential command signals to operate switches, joysticks, etc., via voice, eye movement and gaze tracking, electromyography, electroneurography, electrooculography, electroencephalography, etc. Neuroprostheses typically consist of electrodes that interface with the nervous system and aim to restore lost motor, sensory, or autonomic function. To this end, neuroprostheses can stimulate specific muscles, nerves, or the spinal cord to produce function in end effectors below the level of spinal lesion. Alternatively, the intended movement-related neural activity that is recorded via one of the aforementioned approaches can serve as the control signal for an active orthosis that compensates for the loss of limb function [36]. In this context, neural prosthetic devices that decode intended motion trajectories from the motor cortex to control functional electrical stimulation (FES) devices have been developed [24]. For example, the Freehand System (NeuroControl, Cleveland, OH, USA), shown in Fig. 2, consists of an implantable neuroprosthesis intended to restore hand function in individuals with motor complete injury at the C5/C6 level [59]. It can stimulate specific muscles in the arm and hand in order to produce a useful grip and key pinch. The system consists of a surgically implanted receiver unit incorporating an eight-channel stimulator and electrode combination with an external controller and power supply/microprocessor [31]. Even though it has been shown to positively impact ADL in patients, the Freehand product has been discontinued, primarily due to issues with low market size and high production costs. Neuroprostheses can also stimulate intact peripheral nerves, providing functional restoration of various body organs [24], such as bladder function. In summary, neuroprostheses serve as compensatory functional tools in patients when voluntary movement is impossible due to paralysis. Therefore, this technology has the potential to significantly increase functional quality of life (QOL) in patients with complete SCI and paralysis, and even more so in chronic SCI.
Another recent approach that has contributed to improving SCI therapy is brain–computer interfacing (BCI). These BCIs can record movement-related neural command signals directly from the brain, via intracortically with microelectrode arrays implanted in the motor areas of the brain or extracortically with electrocorticogram or electroencephalogram electrodes. Most of the current BCI designs are based on the principle of recording neural signals and decoding the user’s movement intention to operate an assistive device. BCI has been shown to not only be useful for assistive device control ([34, 49]), but also suitable for rehabilitation due to its therapeutic effects as an adjunct with physical therapy [48, 49]. Therefore, BCIs can be used for neural biofeedback-based training that can assist in rehabilitation as well as act as control mechanisms for neuroprostheses. This dual functionality provides the advantage of using this technology for rehabilitation in patients with acute complete and incomplete SCI to help engage intrinsic neuroplastic recovery mechanisms, and in patients with chronic complete SCI and paralysis. An important caveat to consider in the clinical translation of BCI technologies is that accuracy of decoding movement-related signals has been found to be lower in patients when compared to healthy subjects; further research in developing robust BCI decoding algorithms is necessary [19, 41]. Recently, promising results have been demonstrated in augmenting powered robotic gait assistive exoskeletons with noninvasive electroencephalography (EEG)-based BCIs for direct control by SCI patient user’s movement intents [11, 32]. Such findings suggest that decoding algorithms for BCI-based therapies are improving, and future developments could significantly enhance the use of BCI technologies in rehabilitation of patients with complete SCI.
An alternative to EEG-based BCIs is the use of chronically implanted intracortical, microelectrode arrays to record motor cortical single-unit or multiunit ensemble spiking activity from a person with tetraplegia and use it to control an assistive device such as a robot arm or computer cursor. This approach generally uses the relationship between intended limb movement kinematics and neuronal spiking [39] to decode movement intention [37, 63] in contrast with EEG-based BCIs, which typically map the relationship between whole brain cortical dynamics and movement intention. The intended movement commands are then used to produce corresponding real-time movements in an external device. Ongoing clinical trials are exploring the feasibility of this approach for clinical rehabilitation of tetraplegia due to SCI and other severe movement disorders [10, 26]. Due to challenges posed by the lack of stability of single-neuronal ensemble recordings [45], other approaches have recently explored the use of larger-scale intracortical signals such as local field potentials [20, 28], multiunit activity [55], and electrocorticograms [53, 61]. We refer the reader to Chapter 7 of this book for further information on applications of BCI systems in neurorehabilitation, which further describes current approaches, issues, and applications of this technology.
3.2 Rehabilitation Techniques for Incomplete SCI
Rehabilitation approaches for individuals with incomplete SCI are focused on a combination of compensation, adaptation, and promoting extant neuroplasticity. As a result of these techniques, more than 80 % of individuals with motor-incomplete SCI regain some locomotor function [18]. This can be achieved by activity-dependent plasticity, i.e., an appropriate sensory input can be used to normalize the reflex output of the spinal cord that is disrupted, such as the rhythmic input from a treadmill. Neuroplasticity can also be harnessed by simultaneously training upper and lower limb movements trying to optimize the functional outcome. In the last decade, robotic devices and technology have been integrated into these training programs with promising results [36]. However, depending on the level of the injury, treatments usually apply varied approaches due to differences in rehabilitation goals based on each individual’s need. In the subsequent sections, several rehabilitation approaches based on the severity of injury on ASIA impairment scale (AIS) classification are discussed.
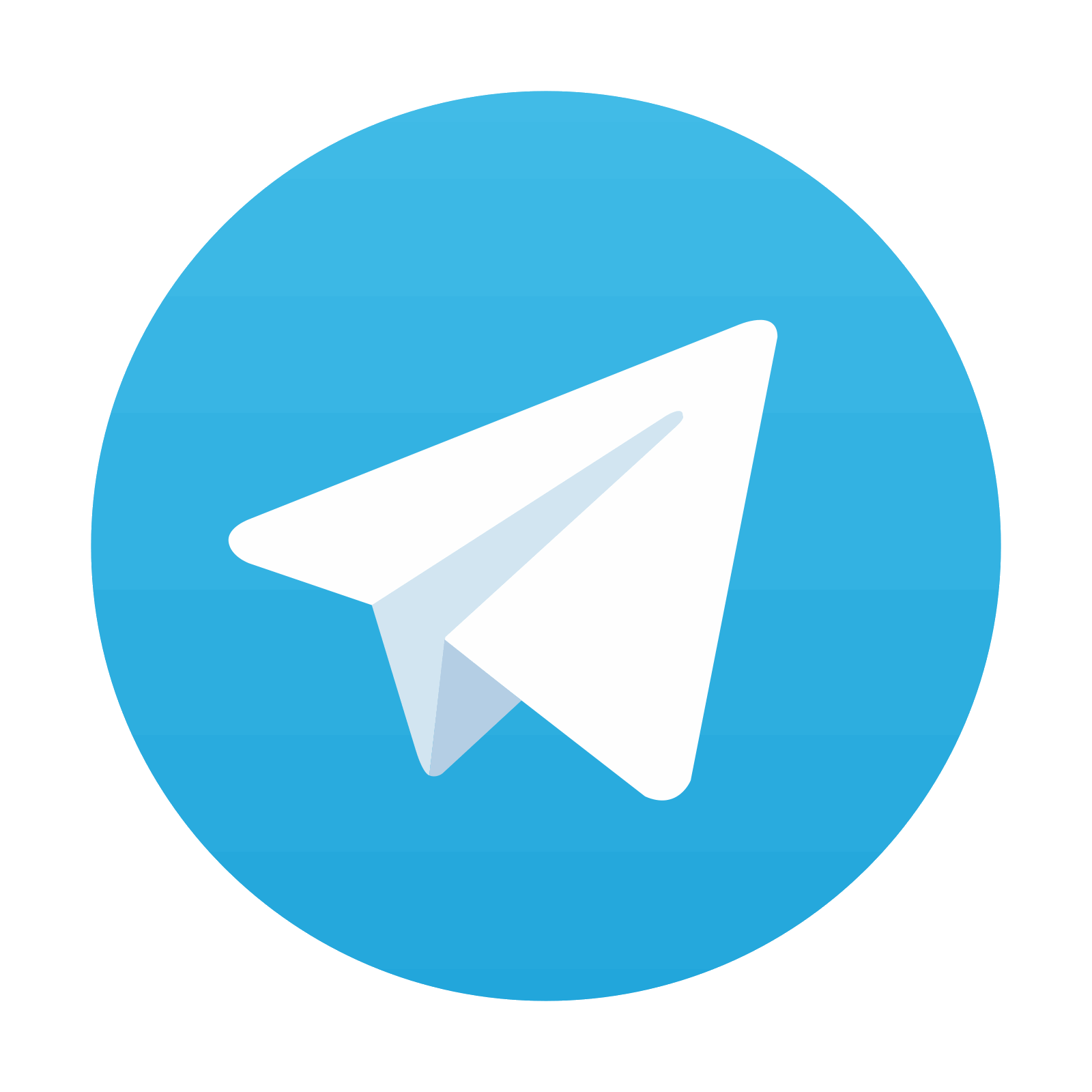
Stay updated, free articles. Join our Telegram channel
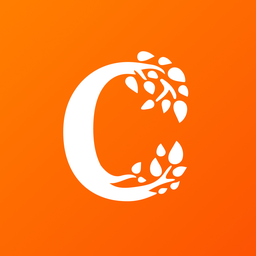
Full access? Get Clinical Tree
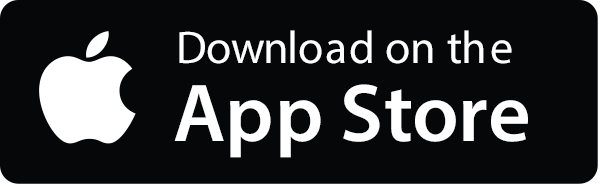
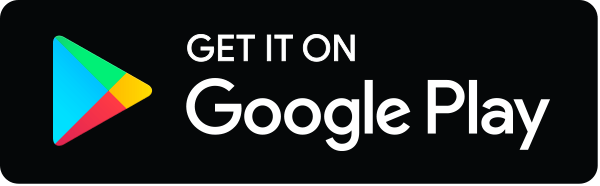