The role of laboratory testing in the diagnosis of neuromuscular disease is described in the first chapter. Tests are ideally used to support a clinically established working diagnosis, not in a random search process. False-positive test results occur with some frequency, and can easily lead to unnecessary testing and interventions as well as potential harm if not measured against pensive clinical analysis.
This chapter will focus on nonhistologic tests that are readily available to most clinicians and potentially useful to the neuromuscular physicians in their assessment of patients. In keeping with the philosophy of this text, emphasis will be placed on tests that have pragmatic application. The science behind the testing will be provided only to the extent necessary to understand the utility, performance, interpretation, and limitations of a test within a given clinical context. The following topics will be addressed:
Electromyography (EMG) and nerve conduction studies (NCS), collectively known as electrodiagnosis (EDX)
Quantitative sensory testing (QST)
Autonomic nervous system testing (ANST)
Routine laboratory (blood) testing
DNA mutational analysis
Biochemical testing for inborn errors of metabolism
Serologic testing
Cerebrospinal fluid (CSF) analysis
Nerve and muscle imaging
Like all tests, EDX has limitations, as do the people who order, perform, and interpret them. The most satisfactory results occur when the requesting physician understands the tests’ value and limitations, and posits specific questions to the electromyographer that the test is capable of answering. A satisfactory result is also dependent on an electromyographer who examines the patient, understands the differential diagnosis of the clinical problem, and tailors the electrodiagnostic examination to adequately explore those possibilities. In keeping with this philosophy, it is readily understandable that the nerves tested during NCS and the muscles selected for EMG, although often guided by algorithm, are frequently modified both on a case-by-case basis both prior to and during its performance.
Attention to detail is important in EDX. One notable example is attention to limb temperature. As a general rule, hand temperatures of >33°C and foot temperatures of >31°C are desirable. Although warm water baths and heating lamps may be used, in our experience, reusable microwaveable heating pads applied to the limbs are the most effective technique for obtaining and maintaining this thermal environment.
With cold limbs, amplitudes of both compound motor action potentials (CMAP) and sensory nerve action potentials (SNAP) are increased. Abnormally low CMAP and SNAP amplitudes could be potentially normalized. Conversely, conduction speeds are reduced, including slowing of conduction velocities and prolongation of distal, F wave, and H reflex latencies (Fig. 2-1). Repetitive stimulation techniques are also affected by limb temperature. Limb cooling diminishes the degree of the decremental response in patients with disorders of neuromuscular transmission (DNMT). Failure to maintain adequate limb or facial temperature could readily lead to a false-negative result. Although it would be unusual for fibrillation potentials to disappear with limb cooling, their prevalence and therefore their detection may be hampered by cool body temperatures as well. In summary, with the exception of cold-induced myotonic discharges in paramyotonia congenita (PMC) and repetitive stimulation techniques in certain muscle channelopathies described below, the accuracy of EDX is improved upon by establishing and maintaining adequate limb warmth of at least 32°C.
Figure 2-1.
Effect of cool limb temperature on motor nerve conduction studies—median CMAPs (compound muscle action potentials) demonstrating factitiously significant increase in distal latency, slightly decreased conduction velocity, prolonged CMAP duration and increased amplitude (note different gain settings) following cooling (A) and corrected by limb warming (B).

Patients and their physicians are concerned about the potential EDX risks in patients who have pacemakers, defibrillators, central lines and altered hemostasis. In general, although testing under these circumstances is not risk free, available published data would suggest that the risk is limited if appropriate precautions are taken. Like most medical decisions, the potential benefits of EDX testing in a patient with any of these situations should be balanced against the risks.
The risk of performing NCS in patients with external wires leading to the heart is unknown but is considered a relative if not absolute contraindication.1 There is a paucity of information as well regarding the risk to patients with central lines, pacemakers, and defibrillators. Both experience and theory suggest the risk is small and nerve conductions appear safe if the stimulus is not delivered in topographical proximity (within 6 cm) to the tubing or wire and a stimulation of 0.2 ms or less is used.2–4 Even less is known about the safety of repetitive nerve stimulation techniques in this setting. A study to address this issue is underway.
Regarding the needle examination and hemostasis, the risk of bleeding or hematoma formation in patients taking anticoagulants or antiplatelet drugs also appears small and is estimated to be approximately 1.5%.5 When present, the risk of clinically significant bleeding also appears to be small. On the other hand, compartment syndrome has been reported in patients with normal hemostasis although its risk is generally considered minimal.6 Available, albeit limited, evidence would support the performance of needle examination in patients who are therapeutically anticoagulated. Caution should be exercised when the international normalized ratio (INR) is supratherapeutic (i.e., >3), platelet count is less than 20,000, or in deep muscles where hematoma formation may not be readily evident or easily compressible. If needle examination is to be done, the smallest diameter that is feasible should be utilized. Needle EMG also poses the risk of pneumothorax, particularly when studying the serratus anterior and diaphragm.4 These muscles, in particular, should be studied by those well versed in anatomy, who are well experienced in the technique, and then only when clinical circumstances warrant. EMG poses a risk to electromyographers as well, largely in the form of the potential transmission of infectious agents through inadvertent needle sticks. The most common preventable reason for these accidents appears to be a hurried and harried examiner who is not adequately attentive.7
Opinions differ regarding the role of clinical assessment in the construction and reporting of the EDX evaluation. Purists believe that EDX conclusions should be based solely on the results of the study and should not be influenced by clinical bias. The argument in support of this philosophy is the potential risk that meaningful EDX observations will be ignored if they do not conform to a preexisting clinical belief. This potential bias is valid and should be considered and avoided by introspective electromyographers. Having said that, it is the strongly held belief of the authors that clinical perspective in the EDX evaluation is integral to the efficient construction and accurate interpretation of the study. There are a number of lines of reasoning to support this perspective.
For example, there are disorders that share identical electrodiagnostic signatures but have differing etiologies, natural histories, and treatment potentials. Early amyotrophic lateral sclerosis (ALS) affecting the lower extremities, polyradiculopathy of severe lumbosacral spinal stenosis or a dural arteriovenous malformation may be electrodiagnostically indistinguishable.8–10 The EDX conclusions in this case should be appropriately weighted by clinical insight. In addition, patients may have more than one disorder affecting the same components of the neuromuscular system. If this is the case, accurate EDX conclusions will be confounded without the clinical insights necessary to distinguish which abnormal EDX parameters are and which are not germane to the problem at hand. A third argument in support of coupling EDX impressions with clinical insight is the realization that in some cases, pathology may be subclinical. It is not uncommon to find mild median nerve conduction slowing across the wrist in individual with a vocation that involves repetitive hand use whose complaints bear no resemblance to the clinical phenotype of carpal tunnel syndrome (CTS). Reporting based solely on EDX result risks unnecessary surgery in patients whose morbidity rests primarily on tendon or joint injury.
With the improvements and uniformity provided by contemporary electrodiagnostic equipment, it can be argued that it is no longer necessary for each laboratory to establish their own normative data. Assuming that attention is paid to accurate measurement, adequate temperature maintenance, and standardized distances for distal latency measurements, normative data provided by a number of reliable published sources are likely to be adequate. It is important however, to recognize the potential pitfalls of population-based “normal” values. Normative data are influenced by age. EDX in infants has to be interpreted by a completely different set of norms than are used in adults. By the same token, conduction studies have to be more cautiously interpreted in the elderly, particularly lower extremity sensory conductions. Although sural and superficial peroneal SNAPs are elicitable in many older patients, they can be absent in otherwise normal patients 60 years of age and older. This may confound the distinction between two common problems in this age group, peripheral neuropathy and lumbosacral polyradiculopathy due to spinal stenosis, the distinction of which relies heavily on evaluation of SNAPs.
Another age-related misinterpretive risk stems from the recognition that larger motor unit potentials (MUPs) may be seen in seemingly normal elderly individuals, particularly in intrinsic hand and foot muscles. This has been attributed to reinnervation resulting from (1) the wear and tear of the process in intrinsic hand or foot muscles, (2) motor unit loss resulting as a normal component of aging, or (3) in response to remotely symptomatic or asymptomatic spondylosis of the lumbar or cervical spine.
There is considerable heterogeneity in normative values for NCS within healthy populations. Any parameter measured may be normal within population based norms but may be differ from the patient’s frequently unknown baseline values. For this reason, focal or unilateral problems are best studied by comparing results with the analogous nerve of the opposite extremity rather than utilizing population norms. In most laboratories, a side-to-side amplitude difference of more than 50% is considered abnormal. Even this represents a potentially insensitive means to detect subtle nerve pathology.
Timing considerations in EDX are critical. In general, it is estimated that complete Wallerian degeneration requires 3–5 days to produce a noticeable decline in CMAP amplitudes, with the nadir occurring between days 7 and 9. Wallerian degeneration in sensory nerves lags slightly behind with amplitude loss becoming apparent between the fifth and seventh days. The SNAP amplitude reaches its lowest point in a monophasic nerve injury by the 10th or 11th day.11 For this reason, an interval of 10 days to 2 weeks between injury and the performance of NCS is ideal in most instances. There is a risk of false interpretation if NCS are preformed prematurely.
This is particularly true with conduction block. In most circumstances, a significant CMAP amplitude above but not below a focal nerve lesion suggests a demyelinating conduction block. This conclusion would implicate a limited number of peripheral nerve disorders with the potential for full and relatively rapid recovery in many cases. If motor conductions are performed hyperacutely within the aforementioned 9-day window before Wallerian degeneration is complete, an axon loss lesion may be falsely interpreted as demyelinating conduction block resulting in erroneous differential diagnostic and prognostic considerations.
The interpretation of the needle portion of the EDX examination is also subject to timing considerations. Fibrillation potentials and positive waves occurring in a muscle at rest, the most sensitive indicator of ongoing denervation on EMG, may develop within days in muscles that are in close anatomic proximity to the site of nerve injury. Three weeks may be required however, for these to develop within all muscles at risk. As many patients may be reluctant to undergo multiple examinations, the EDX should be ideally postponed for 3 weeks after disease onset in most circumstances.
There are at least two circumstances in which it may be preferable to perform EDX earlier than the normal 3-week recommendation. One of these occurs when there is the suspicion or knowledge of a preexisting nerve injury. It may be important for either legal or medical reasons to identify preexisting abnormalities before new ones develop. Performing two examinations, one as early as possible and then a second examination a month or more later, would be best suited to address this issue. A second scenario would be a suspected Guillain–Barré syndrome (GBS) where rapid EDX support for the diagnosis is desired. As in other neuromuscular disorders, it may require days or weeks for the complete EDX signature of GBS to fully develop. Nonetheless, the rapid evolution of NCS abnormalities, even if not diagnostic, in the absence of findings characteristic of other potential causes of acute generalized weakness, can be reassuring to the clinician and guide management decisions in the critical first week of the illness.
EMG is often performed in the evaluation of patients who may eventually undergo muscle biopsy. In order to avoid the potentially confounding variable of needle artifact, it is our practice to restrict the needle examination to one side of the body if muscle biopsy is a consideration. The most appropriate muscle on the opposite side is then recommended to the referring physician. Needle EMG can also elevate serum CK values and potentially introduce another confounding variable in the evaluation of the patient with neuromuscular disease. For this reason, blood should be ideally drawn prior to, immediately after, or greater than 72 hours after EMG performance.12
The routine EMG/NCS examination traditionally consists of motor NCS, sensory NCS, and the needle electromyographic examination. F waves and H reflexes are also commonly tested although in most cases provide complementary rather than novel information. As previously mentioned, nerves and muscles should be selected on a case-by-case basis. Initial selection is based on the diagnostic question posed, the clinical information available, and may be modified as the test unfolds. It is appropriate to emphasize that techniques used to detect DNMT such as repetitive motor nerve stimulation (RNS) testing and single fiber EMG (SFEMG) are not part of the routine evaluation in most laboratories. Once again, the importance of clinical surveillance in test construction is emphasized.
Motor nerve conductions are performed by applying an active surface recording electrode to the midportion of a muscle belly and stimulating the nerve innervating it at one or more locations. The active electrode position is chosen to overly the motor point, that is, the confluence of neuromuscular junctions. This allows for a biphasic waveform known as the compound muscle action potential (CMAP) with a well-defined take off point for accurate measure of latency, waveform amplitude, and area. In addition, a reference electrode is used, placed off the muscle belly and usually on the muscle tendon. The CMAP is obtained by stimulating the nerve in question at anatomically accessible points. To elicit the desired response, the intensity of the electrical stimuli applied to the nerve is increased until all involved axons and muscle fibers are activated and the maximal response is obtained. This is referred to as the supramaximal stimulus and is the desired effect in all routine motor and sensory conduction studies. Each nerve tested may be stimulated at one or more locations, limited only by anatomical accessibility and patient tolerance.
Readily testable motor nerves are the median, ulnar, radial, accessory, facial, tibial, and common peroneal. The phrenic, femoral, axillary, and musculocutaneous nerves can be tested, although in each case technical issues may make reliable and reproducible information more difficult to obtain. The CMAP amplitude of the H response stimulating tibial nerve and recording from soleus represents another motor conduction parameter. The CMAP waveform that is obtained represents the sum of all the individual single muscle fiber action potentials (SFMAPs) within that muscle activated by the nerve stimulus. Because different fibers within a nerve have different conduction velocities, the waveform is dome like rather than spiked in its configuration. The proximal or left-hand side of the waveform represents the action potentials of the fibers innervated by the fastest conducting axons. The trailing aspect of the dome represents the action potentials of the muscle fibers innervated by the slowest conducting motor axons (Fig. 2-1). As stimuli are delivered at increasing distances from the target muscle, that is, more proximal locations, the distance between the initial and terminal aspects of the CMAP waveform widens. This results in an increasing duration of the CMAP waveform without a reduction in the area under the curve, the number of activated nerve and muscle fibers being identical. This is the basis of the normal physiologic waveform dispersion described below.
Typically, three parameters are measured with an additional parameter assessed more subjectively. The baseline to peak amplitude and the area under the curve of the CMAP waveform are proportionate to the number of viable muscle fibers that are activated within the recording radius of the active recording electrode. These parameters represent an indirect measure of the number of viable and excitable axons that innervate them. Reduction in CMAP amplitude results from axon loss anywhere between anterior horn cell and neuromuscular junction, impaired neuromuscular transmission (particularly presynaptic), or of loss of muscle. In some instances, the CMAP amplitude may be adversely affected by diseases that preferentially affect the integrity of the myelin sheath producing conduction block or temporal dispersion. This will be described subsequently.
The other two parameters routinely measured are distal latency (time between stimulus delivery and lead edge of CMAP and conduction velocity. These parameters are measures of conduction speed and therefore primarily reflect myelin integrity. Conduction velocity and distal latency are reported separately in motor conduction studies for a number of reasons. The distal latency reflects conduction along different segments of nerve (wrist to hand or ankle to foot) than the conduction velocity (elbow to wrist or knee to ankle). In certain pathologic conditions, one parameter may be abnormal whereas the other remains unaffected. Distal latency and conduction velocity are also reported differently for purposes of technical accuracy. Distal latency measures not only nerve conduction but neuromuscular transmission time as well. In addition, terminal nerve twigs attenuate in diameter and have a conduction velocity that does not accurately reflect conduction speed in the more proximal nerve.
The last parameter to be assessed on a more subjective basis is CMAP appearance. Although morphologic changes can be measured by comparing ratios of CMAP duration to amplitude, these are usually made on a qualitative rather than quantitative basis. Subtle changes may occur in normal individuals when CMAPs are obtained from stimulation at different points along the course of a nerve. As previously described, this is referred to as physiologic dispersion. With physiologic dispersion, it is estimated and modeled that the CMAP amplitude with proximal stimulation should never drop below 80% of that obtained from the most distal stimulus site. Nerve root stimulation sites provide the notable exception to this rule.13 More dramatic reductions of CMAP amplitude, particularly over short segments of nerve, suggest demyelinating pathology due to conduction block or temporal dispersion, anatomic variants such as a median to ulnar crossover, or alternatively technical error (Figs. 2-2A,B and 2-3).
Figure 2-2.
(A) Short segmental incremental stimulation in normal individual demonstrating identical CMAP (compound muscle action potential) waveforms with equivalent spacing between consecutive waveforms and (B) ulnar neuropathy at the elbow with focal demyelination with conduction block (amplitude reduction between responses 3 and 4), focal slowing (widening baseline interval between responses 3 and 4), and mild temporal dispersion (increased CMAP duration between responses 3 and 4).

CMAP afterdischarges represent one other potential alteration of CMAP morphology. When present, these repetitive CMAPs follow single or repetitive supramaximal nerve stimuli. They appear as one or more additional negative peaks in the immediate aftermath of initial CMAP detectable either with routine motor conductions, with repetitive stimulation or with F wave assessment. The afterdischarges may actually interfere with F wave identification. Afterdischarges are not uniform with consecutive stimuli and have much smaller amplitudes than the initial supramaximal response (Figs. 2-4 and 2-5). They are uncommon, typically identified in disorders of nerve hyperexcitability in which nerve or muscle depolarization persists or repolarization is delayed (Chapter 10). They are commonly associated with continuous motor unit activity at rest with needle EMG. There is a spectrum of these spontaneous discharges ranging from single random MUP discharges (i.e., fasciculation potentials), to doublets or other multiplets which when discharging rhythmically or semirhythmically appear as myokymic discharges. At the extreme of these spontaneous discharges are high-frequency decrescendo waveforms known as neuromyotonic discharges. The generators of these discharges are believed to reside within motor nerve, probably within terminal twigs.
The clinical value of afterdischarge identification is derived from their specificity. They are associated with a limited number of disorders that may affect nerve, neuromuscular transmission, or muscle. Neuromyotonia, also known as Isaac syndrome or the syndrome of continuous muscle fiber activity is the most notable form of nerve hyperexcitability.14–17 Our current understanding implicates malfunction of nerve potassium channels by antibodies directed against contactin-associated protein-like 2 (Caspr2). Presumptively, the afterdischarges result from prolonged nerve depolarization due to impaired potassium channel function resulting in the inability of nerves to rapidly repolarize.
In addition, afterdischarges may occur in disorders in which there is prolonged cholinergic activity at neuromuscular junctions such as toxic exposures to organophosphates, anticholinesterases or congenital acetylcholinesterase deficiency, and slow channel syndrome.18 These afterdischarges appear similar to those that occur in disorders of nerve hyperexcitability in that they occur following a single supramaximal stimulus delivered to a motor or mixed nerve. The physiologic basis for these afterdischarges appears to be prolongation of the end-plate potential at the neuromuscular junction, unrelated to the delayed neurotoxic effects that frequently occur with toxic organophosphate exposure.
A different type of afterdischarge of muscular rather than nerve origin referred to as post-exercise myotonic potentials (PEMPs) may occur with myopathy associated with impaired sodium channel, particularly PMC, and to a lesser extent chloride channel function, that is, myotonia congenita (MC).19,20 PEMPs are not seen in sodium or calcium ion channel disorders that produce periodic paralysis phenotypes.19 These afterdischarges can be differentiated from those of neural origin as they do not occur after a single supramaximal motor stimulation but only in the context of the short exercise testing that is described below. They persist if repetitive stimulation is delivered immediately post-exercise but dissipate as the interval between exercise and stimuli evolves, whether or not the stimuli are repetitive or individual.
Sensory conduction studies are also performed with surface electrodes in most instances. Unlike motor conductions, the recording electrodes are placed over nerve not muscle. Nerve rather than muscle action potentials are measured, with maximal amplitudes measured in micro- rather than millivolts: making them more technically difficult to obtain. The resulting wave form is referred to as an SNAP. The same disc recording electrodes, or in the case of the median and ulnar nerves, ring electrodes on digits are utilized. The tested nerve is then stimulated at either a more proximal or a distal location than the recording site. The former technique is described as antidromic, as the impulse travels in the direction opposite to that of normal centripetal physiologic conduction in sensory nerve fibers. With stimuli delivered distal to the recording site in sensory or mixed nerves, conduction is considered orthodromic. Nerves routinely studied include the median, ulnar, dorsal cutaneous ulnar, radial, medial antebrachial cutaneous, lateral antebrachial cutaneous, sural, and superficial peroneal. The lateral femoral cutaneous, saphenous, posterior cutaneous nerve of the forearm, medial and lateral plantar nerves, and median and ulnar studies with less commonly used digits are less frequently tested. Many of these latter studies are fairly easy to obtain in the young, healthy, slender, and nonedematous but can be technically difficult in those with the opposite characteristics.
In many laboratories, only two parameters are measured; SNAP amplitude and either distal latency or conduction velocity (Fig. 2-6). As there are no neuromuscular junctions to contend with in sensory nerves, both the distal latency and the conduction velocity are measures of nerve conduction speed, differing only in the segment of nerve tested and the units with which it is reported. With motor conductions, there are some disorders in which distal latencies are prolonged disproportionate to forearm or leg conduction velocities. As there are few, if any, recognized conditions in which conduction speed is consistently more affected in one segment of sensory nerves than another, it can be argued that the reporting of distal latency as the sole measurement of sensory conduction speed is adequate. With motor distal latencies, the onset of the waveform is used for measurement, thereby identifying the fastest conducting axons. With SNAPs, the distal latency is typically measured from the waveform peak rather than the onset. This is done for technical reasons, as the onset of the SNAP waveform may be difficult to reproducibly identify. Sensory conduction velocities are however measured utilizing the SNAP takeoff from baseline rather than peak.
Figure 2-6.
Sensory nerve action potentials in a normal individual demonstrating normal physiologic dispersion over distance with decreased amplitude and prolonged duration of median SNAP (sensory nerve action potential) waveform stimulating at elbow (bottom) as compared to stimulating at the wrist (top).

The second, and in the majority of situations, more important SNAP parameter is amplitude. As SNAPS are nerve rather than muscle action potentials, amplitude reduction does not occur on the basis of impaired neuromuscular transmission or myofiber atrophy/loss. With the exception of certain types of demyelinating pathology described below, reduced SNAP amplitude indicates either advanced age, excessive subcutaneous tissue or fluid, poor technique, or, most commonly, loss of peripheral sensory axons; the pathology occurring anywhere at or distal to the dorsal root ganglia.
Detecting morphologic change of SNAP waveforms is of limited value. Waveforms obtained with proximal stimuli are typically significantly reduced in amplitude and prolonged in duration in comparison to their distally obtained counterparts (Fig. 2-6). This is due to the same principle of physiologic dispersion described above. This phenomenon is more pronounced than its motor counterpart due to the far wider range of conduction velocities in sensory nerves. As a result, attempts to identify demyelinating conduction block or significant morphologic differences in SNAP waveforms are not routinely attempted, particularly with stimuli delivered at wide interval distances.
Motor and sensory conduction studies are typically performed in the below elbow and knee segments where nerves are more anatomically accessible. F waves and H reflexes have potential value as a result of their ability to assess conduction in more proximally located nerve segments. F waves can be obtained by delivering supramaximal stimulation to any motor or mixed nerve in a normal individual, in most instances. F waves can be difficult to obtain in certain nerves, for example, common peroneal, even in the apparent absence of pathology. For that reason, it can be perilous to suggest the existence of nerve injury based solely on the absence of an F response from a single nerve. In normal adults, H reflexes can be elicited from the soleus muscle while stimulating the tibial nerve and, on occasion, from the flexor carpi radialis. Identification of H reflexes in other nerve/muscle pairs implies the existence of upper motor neuron disease due to decreased central nervous system inhibition on the reflex arc, analogous to a hyperactive deep tendon reflex.
The relevant anatomy and physiology of an F response can be described in the following manner. When a supramaximal stimulus is delivered to a nerve, the primary orthodromic response is the CMAP (M wave) as previously described. In addition, the initial nerve depolarization also produces an antidromic action potential traveling centripetally toward the spinal cord. At the level of the corresponding anterior cell(s), this antidromic action potential establishes a persistent or second action potential at the level of either the perikaryon or its axon hillock. This supplemental action potential is carried in a centrifugal or orthodromic direction along the entire length of one or more of the same motor axon(s) back to the original target muscle. As a result, the muscle is depolarized twice in response to a single stimulus, the second muscle action potential (F wave) having understandably a much longer latency and smaller amplitude. Unlike the initial CMAP, which represents the action potentials all of the responsive muscle fibers, each (F) response represents the action potentials of muscle fibers belonging to a single motor unit. With each sequential stimulus, different motor units are typically activated. As a result, sequential F wave responses have varying latencies and morphologies in comparison to those occurring with the previous or subsequent stimuli.
F waves have significant limitations. Even in a normal nerve/muscle pair, F waves do not result from each stimulus. Amplitude measurements are of no particular value, as these represent the action potentials of only a small and varying proportion of single muscle fibers. There is heterogeneity of F wave latencies as sequential responses rarely arise from motor axons with identical conduction velocities (Fig. 2-7). A number of latency measurements can be made, the response with the shortest latency typically being the parameter reported. The potential value of F waves is their ability to detect conduction slowing over the segments of nerve not tested by routine conduction velocity measurements, that is, the proximal to elbow and knee segments. This value is most apparent early in the course of acquired demyelinating neuropathies, where prolonged F wave latencies may occur because of disease predilection for nerve roots, prior to slowing of conduction velocity or prolongation of distal latency in more distal nerve segments. In most cases however, F waves are either absent, or prolonged in the setting of slowed conduction velocities. Simultaneous slowing of conduction velocities and F waves has no localizing value, as the slowing of the F latency in this circumstance may represent slowing in the same distal aspect of the nerve where the conduction velocity is measured.
Figure 2-7.
F waves—11 consecutive supramaximal stimuli delivered to the median nerve at the wrist in a normal individual while recording from the abductor pollicis brevis muscle demonstrating uniform CMAPs (compound muscle action potentials) but typical F wave behavior, that is, waveforms that are variable in occurrence, latency, and morphology.

The H reflex represents the electrophysiologic analog of the Achilles deep tendon reflex. As in the F response, the stimulus applied to the tibial nerve in the popliteal fossa will travel in two directions. Unlike F waves, the H reflex is obtained with submaximal stimulus intensity. With delivery of stimuli of low intensity and long duration (1 ms), the lower-threshold 1 A sensory fibers within the tibial nerve are preferentially activated. As a result, the initial action potentials are propagated solely within thickly myelinated sensory nerve fibers. These impulses travel both centrifugally, where they have no known clinical or diagnostic consequence, and centripetally (orthodromically) along tibial and sciatic sensory fibers. Impulse transmission through the dorsal root of the S1 segment allows completion of a monosynaptic reflex to S1 anterior horn cells. Activation of these produces the H reflex representing a long latency CMAP originating from the soleus muscle. Typically, the H reflex has a latency in the high 20 to mid-30 μs range, depending on patient height.
As the intensity of the stimulus delivered to the tibial nerve increases, characteristic H reflex behavior is demonstrable. Action potentials will develop within the higher-threshold tibial motor fibers in addition to the 1 A sensory fibers already activated. These motor nerve action potentials also travel bidirectionally. The orthodromic impulses will activate the soleus muscle producing a typical CMAP. This has a far shorter latency than the H reflex and does not typically make its appearance until the H reflex is well established. The antidromic action potentials created in tibial motor fibers have a different effect. These will collide in a proximal location with the action potentials responsible for the H reflex. As the stimulus intensity increases, more tibial motor axons are depolarized resulting in increasing soleus CMAP amplitude but declining H reflex amplitude. Eventually, the H reflex will disappear while the CMAP will become supramaximal.
In summary, in response to sequential stimuli of 0.5–1-ms duration delivered to the tibial nerve in the popliteal fossa with increasing intensities, the H reflex appears first. Subsequently, the CMAP or M wave appears and enlarges to its supramaximal amplitude while the H reflex declines in amplitude and disappears. The tibial motor fibers distal to the stimulus site are depolarized twice, whereas both the tibial sensory fibers and the tibial motor fibers proximal to the stimulation site are depolarized once in response to a single stimulus (Fig. 2-8).
Figure 2-8.
H reflex—five consecutive and increasing stimuli to the tibial nerve in the popliteal fossa in a normal individual, recording from the soleus demonstrating a typical H reflex pattern, that is, H reflex amplitude initially > M response amplitude, subsequent peaking than decline, and eventual absence of H reflex, associated with gradual increase to supramaximal M response. Note uniform H reflex latency.

Both the maximal amplitude and the latency of the H reflex can be measured. The former estimates the number of viable motor units and muscle fibers within the S1 segment/soleus muscle complex and is typically greater than 1 mV in size. The latter provides at least an estimate of conduction speed within the motor and sensory fibers of the S1 segment. As in the case of F waves, H reflexes have greatest utility and localization value when these are abnormal in the setting of normal routine conduction parameters. This applies most frequently early in the course of acquired demyelinating polyneuropathies. In addition, H reflex amplitudes have value in the assessment of S1 radiculopathies. If an H reflex is absent more than a week after symptom onset in the setting of normal routine conduction parameters and reduced recruitment in S1 innervated muscles, proximal conduction block in the tibial nerve, sciatic nerve, sacral plexus, or S1 nerve root can be inferred. Focal slowing of nerve conduction in a proximal location is theoretically detectable by H reflex assessment. In reality, this slowing is usually obscured by normal conduction speed in the other normal and more extensive parts of the S1 reflex arc. Attempts to provide an anatomic diagnosis of a focal neuropathy or radiculopathy on the basis of by F and H responses alone should be discouraged.
Performance and interpretation of RNS techniques evolves from an understanding of the normal physiology of neuromuscular transmission. Muscle end-plate potentials (EPPs) are the precursors of muscle fiber action potentials. Unlike nerve or muscle action potentials that are all or none events that precede and follow EPPs respectively, EPPs are graded. Their amplitudes are proportionate to the number of successful interactions between acetylcholine (Ach) molecules and muscle end-plate receptor sites. Quantal release of ACh decreases with successive stimuli delivered in intervals of greater than 200 μs (e.g., <5 Hz). Under normal circumstances, this quantal and resultant EPP decline is of no practical importance, as there is a considerable physiologic reserve. With disease states that alter either the presynaptic release of ACh or postsynaptic receptor responsiveness, this reserve becomes inadequate. The EPP at individual neuromuscular junctions may fall below the threshold for myofiber action potential generation, and both CMAP amplitude and muscle strength may decline. As a result, slow (2–5 Hz) repetitive stimulation may produce a successive decline or decrement in CMAP amplitude in both pre- and postsynaptic (Fig. 2-9) DNMT. To avoid false-positive results based on technical factors, a decrement of at least 10% is required to be considered abnormal although a smaller decrement in a technically pristine study is suspicious. This decremental response has both clinical and single fiber analogues, that is, fatigable weakness and blocking of single fiber action potentials, respectively.
The recording and stimulating electrode placement in RNS is identical to routine motor conductions. Only the manner of stimulus delivery is altered. As mentioned above, the diagnostic yield of RNS will improve by ensuring that the tested muscle is warm and by removing drugs that augment neuromuscular transmission such as pyridostigmine prior to testing. RNS often produces unwanted movement artifact, which may require limb immobilization in order to secure a technically reliable study. Unfortunately the muscles with the highest diagnostic yield are also those most prone to movement and technical artifact.
False-positive decremental responses due to technical error are commonplace. In order for a decremental response to be considered pathologic, it must be reproducible and conform to the typical pattern. This pattern is best understood by understanding its physiologic basis. Pathologic decrements are not linear. Typically, the CMAP decline between two consecutive responses is greatest between the first and second stimuli and reaches its nadir by the fourth response. The reason for this latter phenomenon is the mobilization of ACh stores in the presynaptic neuron that allows delayed restoration of the immediate release pool. This allows for partial augmentation of the EPP after the fourth or fifth consecutive stimulus. With subsequent stimuli, the CMAP amplitude then begins to increase slightly although it never reaches the size of the initial response. If a train of 8–10 stimuli are delivered, the resulting configuration will have an asymmetric saucer-like appearance, with the left edge being higher. This configuration is of particular importance in distinguishing variation in CMAP amplitude due to disease from that due to technical considerations which are commonplace and easily misinterpreted by the unwary.
The electrophysiologic basis of the incremental response is closely related to calcium’s role in the presynaptic release of ACh. When presynaptic ACh release is impaired by disease, it can be enhanced by augmenting the concentration of calcium in the presynaptic terminal. In a seemingly paradoxical manner, this can be accomplished by the delivery of repetitive stimuli at a frequency of greater than 5 Hz. Stated in a different way, this is accomplished with stimuli delivered at intervals shorter than 200 μs. In presynaptic DNMTs, the baseline CMAP is typically reduced, at times dramatically. This is the most notable difference between pre- and postsynaptic disorders and sets the stage for the ability to demonstrate an incremental response. The initial low amplitude CMAP can be increased by a factor of 100% or more by either “fast” repetitive stimulation (5–50 Hz) or more humanely by brief exercise of the muscle being studied (Fig. 2-10A and B). With the brief exercise technique, a supramaximal stimulus is followed by 10 seconds of isometric exercise to the muscle being studied, and then immediately by a second post-exercise supramaximal stimulus. Trains of fast repetitive stimulation are typically reserved for those who cannot perform or cooperate with the post-exercise technique.
Figure 2-10.
Incremental response to brief (10 seconds) exercise in patient with LEMS (A) (trace 1 ulnar CMAP at baseline stimulating at wrist, trace 2 ulnar CMAP immediately after 10 seconds of isometrically resisted finger abduction stimulating at wrist, trace 3 ulnar CMAP 1 minute later stimulating at elbow). Incremental response to 20 Hz fast repetitive stimulation (B). CMAP, compound muscle action potential; LEMS, Lambert–Eaton myasthenic syndrome.

An incremental response is defined by a >100% increase in CMAP amplitude comparing the post-exercise response to the baseline. The degree of increment cannot exceed the difference between the baseline and premorbid CMAP amplitude for that muscle. A physiologic increment (i.e., higher amplitude, shorter duration, identical area under the curve) may occur in normal patients but typically does not exceed 40% of the baseline. It implies a more synchronous discharge of the component SFMAPs that constitute that CMAP waveform.
The electrodiagnostic approach to a patient with a suspected DNMT is dependent on the clinical context and on the initial, supramaximal CMAP amplitude. To overly simplify the concept, if it is small, try to make it bigger, and if it is big, try to make it smaller. In other words, in the case of either a reduced initial CMAP amplitude or a decrement in response to slow repetitive stimulation, an attempt to increase the CMAP amplitude is made. This can be accomplished by brief exercise, fast repetitive stimulation (10–50 Hz) or by administration of an anticholinesterase medication. Conversely, when faced with a normal initial CMAP amplitude and a suspected DNMT, the electrodiagnostician should seek to produce a decrement by slow repetitive stimulation without, or if necessary, with 1 minute of isometric exercise.
To elaborate, presynaptic DNMT have electrophysiologic properties that are both shared and distinctive in comparison to their postsynaptic counterparts. In suspected myasthenia gravis (MG), the initial CMAP amplitude is typically normal. The first step is to try and demonstrate a decremental response to slow repetitive stimulation. Ideally, for the sake of efficiency, RNS would be performed on a clinically weak muscle that has been warmed. The absence of decremental response to slow repetitive stimulation in a weak muscle would effectively preclude the diagnosis of autoimmune MG as the cause of that weakness. If a decrement is demonstrated, the train of stimulation can be repeated following 10 seconds of exercise to look for post-exercise facilitation or decrement repair. If a decrement is not demonstrable at baseline, repeating the train once a minute for 5 minutes following 1 minute of exercise applied to that muscle may improve diagnostic yield. This phenomenon is referred to as post-exercise exhaustion.
Presynaptic DNMT also decrement with low rates of repetitive stimulation. Early in the course, this may be the only abnormality found with nerve conductions. Presynaptic DNMT are usually distinguished from MG electrodiagnostically on the basis of low baseline CMAP amplitudes. In this case, the initial response from the electrodiagnostician should be to attempt to elicit an incremental response. This is easily done in a cooperative patient by exercising the appropriate muscle for 10 seconds and then immediately delivering a second supramaximal stimulus as described above. If the patient is not cooperative, a train of fast repetitive stimuli (10–50 Hz) may be used as a surrogate. If an increment is demonstrated, a subsequent train of stimuli delivered at 2–3 Hz will produce a characteristic decrement and further solidify the diagnosis of a neuromuscular transmission defect.
The nondystrophic muscle channelopathies constitute a complex, overlapping group of disorders related to gene mutations of chloride, calcium, sodium, and potassium (Andersen–Tawil syndrome) channels in muscle. They will be described in detail in Chapter 30. As a result of their pathophysiologies, the phenotypes are typically dominated at least initially by episodic symptoms, either stiffness related to myotonia, weakness related to periodic paralysis, or a combination of both. Stiffness is felt to result from persistent muscle fiber depolarization and contraction whereas periodic weakness is felt to represent a more severe degree of depolarization rendering the muscle inexcitable. In chronic stages, particularly in the periodic paralyses, persistent weakness may develop.
Electrophysiologic testing of the nondystrophic myotonias involves detection of afterdischarges known as PEMPs as previously described in both routine motor conductions and F wave determinations, the presence or absence of myotonic discharges on needle EMG, response to repetitive nerve stimulation, and in particular, responses to short- and long-exercise testing which will be the focus of this section. Short- and long-exercise tests offer diagnostic support for both the existence and type of muscle channelopathy.19,21 The tests are variations of standard motor NCS which utilize the ulnar or common peroneal nerves recording from the abductor digiti minimi or extensor digitorum brevis muscles, respectively. Both forms of exercise testing require careful attention to uniform patient positioning, limb temperature, muscle relaxation, and stimulus intensity.
Both short- and long-exercise tests are performed by maintaining limb temperature at 32–34°C and establishing a stable baseline, supramaximal CMAP amplitude. With the short-exercise test, a single supramaximal stimulus is delivered, the tested muscle is isometrically exercised for 10 seconds, followed by an immediate post-exercise supramaximal stimulus and six additional single stimuli every 8 seconds are delivered over the course of the next 50 seconds. After a 10-second rest period between trials, two subsequent, identical trials are performed, each preceded by 10 seconds of isometric exercise. Five patterns of abnormality have been described, the first three of which utilize the short-exercise test alone. The other two patterns are defined by a combination of the long- and short-exercise tests.
In normal individuals, there is a slight CMAP amplitude and/or area increment immediately following exercise that rapidly returns to baseline during the first trial and does not differ with the second and third trials.19 The CMAP amplitude rapidly returns to baseline by the second or third stimulus of the first trial (Fig. 2-11).19,21 The mean increment in immediate post-exercise CMAP amplitude is 4% (range −28 to +27) and the mean increment of CMAP area is +3% (range −58 to +84).21 An increment of <10% or decrement <20% in CMAP amplitude and/or area compared to baseline is considered normal.21 The short-exercise test has been further refined by using the same algorithm following limb cooling and rewarming, utilizing a combination of both amplitude and area to improve both the sensitivity and specificity of increment or decrement measurements.21 Cooling is typically delivered for 7 minutes with a target cutaneous temperature of 15°C. In normal individuals, cooling with or without rewarming the limb does not alter the normal pattern.20,21
The long-exercise test is performed with identical electrode application.21 The muscle to be studied is isometrically exercised for 5 minutes. During the period of exercise, single supramaximal stimuli are delivered at 1-minute intervals. Subsequent to the exercise, single supramaximal stimuli are then delivered immediately, every minute for 5–6 minutes, and then every 2–5 minutes for 40–50 minutes. The long-exercise test is of the greatest utility in the identification of the periodic paralyses. Normal patients may demonstrate a minimal initial decrement in CMAP amplitude and/or area with a rapid return to baseline (Fig. 2-12). In African-Caribbean controls the mean decrement may be slightly greater. Authorities recommend that an abnormal decrement on long-exercise testing for amplitude and area exceeds 40%.21
The response patterns with repetitive stimulation seen in the dystrophic or nondystrophic myotonic disorders differ from those seen in pre- and post-synaptic DNMT. In general, because of discomfort, repetitive stimulation is performed in suspected nondystrophic myotonia cases only when the remainder of the electrodiagnostic assessment is inconclusive. The major value of repetitive stimulation in this setting is increased sensitivity, particularly in recessively inherited MC.22 In the nondystrophic myotonias, there is typically no decremental response prior to exercise. Depending on the specific disorder, and the frequency of repetitive stimulation, there may be either a decrescendo pattern in which the CMAP amplitude continuously declines (10-Hz stimulation in a warm limb in MC, or 10-Hz stimulation following limb cooling in PMC) or a pattern in which there is an initial dramatic reduction in CMAP amplitude following exercise which then declines further and then gradually increases with 3-Hz stimulation in PMC) (Fig. 2-13).19,22,23
The following is a summary of the typically patterns of abnormalities seen in MC phenotype associated with chloride channel mutations, PMC, potassium-aggravated myotonia (PAM), sodium channel myotonia (SCM), and hyperkalemic periodic paralysis (hyperKPP) associated with sodium channel mutations, and hypokalemic periodic paralysis associated with both calcium (hypoKPP1) and sodium channel (hypoKPP2) mutations. The reader is referred to Table 2-1 and Chapter 30 for summary and complete description of these disorders.
Phenotype | Channel | Short-Exercise Effect on CMAP Amplitude | Post-Exercise Myotonic Potentials | Response to Repeat Short Exercise | Response to Cooling and Repeat Short Exercise | Long Exercise Effect on CMAP Amplitude | Myotonic Discharges on EMG | Other Disorders in Which This Pattern Can Be Identified |
---|---|---|---|---|---|---|---|---|
MC (type 2 pattern) | Cl– | Immediate ↓ with subsequent repair | Yes | Less prominent CMAP ↓ | 1. AR – no change 2. AD- exaggerated ↓ CMAP amplitude | No change | Yes | 1. PAM 2. MD 1 & 2 |
PMC (type 1 pattern) | Na+ | Immediate ↓ but less than type 2 but with longer effecta | Yes | More prominent ↓ CMAP amplitude | Even further ↓ CMAP amplitude | Prolonged and significant ↓ amplitude | Yes | None to date |
Other sodium channel myotonias (type 3 pattern) | Na+ | No change | No | No change | Variable | No change | Yes | None to date |
HyperKPP (type 4 pattern) | Na+ | Immediate ↑ that persists | No | Further ↑ CMAP amplitude | Unknown | ↑ amplitude immediately that ↓ over time | Yes (not universal) | HypoKK-2 |
HypoPP-1 (type 5 pattern) | Ca++ | No change | No | No change | Unknown | ↓ amplitude immediately that ↓ further over time | No | HypoKK-2 |
HypoPP-2 (type 4 or 5 patterns) | Na+ | Type 4 or 5 | No | Type 4 or 5 | Unknown | Type 4 or 5 | No | HyperKPP HypoPP-1 |
In MC with short-exercise testing, CMAP amplitude and area decrement is the greatest in the initial response following exercise (Figs. 2-14 and 2-15). If it exceeds 40% of baseline, it is considered pathognomonic of a chloride channel disorder.21 With the next six stimuli delivered over the ensuing 50 seconds, the decrement lessens and the CMAP amplitudes and areas gradually approach baseline thus rendering a curve with an ascending positive slope. With the subsequent two trials, the magnitude of the decrement lessens but the trajectory of the curves remains the same. With cooling, the magnitude the decrement increases slightly, particularly in dominantly inherited disease. Although this type 2 pattern is seen with both recessively and dominantly inherited forms of MC, the decrement is far more dramatic in the former (Fig. 2-14).20 This pattern is seen in approximately 83% of patients with confirmed chloride channel mutations with traditional amplitude comparisons but improves to 100% if amplitude and area comparisons are done concordantly 19,21 PEMPs in the short-exercise test in response to single or repetitive stimuli are found in approximately one-third of individuals with MC. Myotonic discharges with EMG are found in essentially all patients with MC.
A type 2 pattern is seen predominantly in patients with MC but has been identified in sodium channel mutations associated with PAM, hyperKPP with myotonia, and both myotonic muscular dystrophy types 1 and 2.19,20 In myotonic muscular dystrophy, the short-exercise testing with and without cooling produces a similar pattern of lesser magnitude than recessively inherited MC. Long-exercise testing in MC patients produces a pattern indistinguishable from controls.
Short-exercise testing in PMC produces a different configuration. There is little or no decrement in CMAP amplitude and area immediately following exercise. With the subsequent six stimuli however, the amplitude increasingly declines, providing a curve with a negative rather than positive slope. The magnitude of this response becomes more dramatic in the second and third trials. In this type 1 pattern, the most dramatic effect occurs following limb cooling, or rewarming following cooling.19,21 Although the configuration of the curves remains the same, the magnitude of CMAP amplitude and/or area decrement is even more dramatic. A CMAP amplitude and area decrement of >20% in response to cooling with or without rewarming is thought to be pathognomonic of PMC21 (Fig. 2-16).
Post-exercise myotonic potentials in response to single or repetitive stimuli following brief exercise are found in essentially all PMC patients. They dissipate both within an individual trial and between subsequent short-exercise trials. Pattern 1 has been exclusively associated with the PMC phenotypes/genotypes to date. The result of long-exercise testing in PMC also differs considerably from MC. A significant and persistent CMAP amplitude and area decrement occurs averaging a 66% reduction in comparison to baseline. The clinical weakness associated with the long-exercise test may preclude its completion. Myotonic discharges with EMG are anticipated in patients with PMC.
One specific sodium channel gene mutation resulting in the PMC phenotype (Q270 K) has an apparent unique short-exercise test signature.20 Short-exercise testing without cooling demonstrates a type 2 pattern essentially identical to that seen in MC, that is, an initial CMAP amplitude and/or area decrement immediately post-exercise that improves within the first trial and then between subsequent trials. After limb cooling, the pattern reverts to approximate the type1 pattern typically seen in PMC, that is CMAP amplitudes and/or areas decrement with a downward slope within the first trial that worsens with each of the subsequent two trials.
In most PAM and other myotonia/periodic paralysis syndromes associated with other sodium channel mutations, short-exercise testing is essentially normal although cold-induced reduction in CMAP amplitudes have been described in at least two genotypes.20 PEMPs are rarely described in PAM and the SCMs, disorders that clinically overlap PMC and MC.19,21 There is no significant CMAP amplitude and area decrement with short exercise, either prior to or subsequent to limb cooling.19 This has been referred to as the type 3 pattern (Fig. 2-17). No significant change in CMAP amplitude or area is seen in response to long-exercise testing in PAM. Myotonic discharges with EMG are anticipated in these disorders.
In hyperKPP, different authors describe different short-exercise test results. Fournier et al. describe an immediate CMAP amplitude and area increment that exceeds that seen in controls both in amplitude and in duration of effect (Fig. 2-18).19 It persists throughout the entire minute of the study. With repetitive trials of short exercise, the CMAP amplitude and area increments in comparison to baseline by an average of 64%.19 According to Tan et al. however, short-exercise testing does not differ from normals in either hyperKPP or hypoKPP-1 patients.21
Distinguishing between hyperKPP and hypoKPP electrophysiologically may be either relatively easy or hard depending on the response to short-exercise testing and the presence or absence of myotonic discharges. According to Fournier and colleagues, the type 4 pattern is characteristic of hyperKPP or some hypoKPP-2 patients (Fig. 2-17). It is defined by occasional myotonic discharges in some hyperKPP patients, an absence of PEMPs, an incremental CMAP amplitude pattern in short-exercise testing as described in the previous paragraph, and the long slow decrement in response to long-exercise testing.19 A decrement of at least 40% of CMAP amplitude and area with long-exercise testing is found in a majority of periodic paralysis patients and may be seen in Andersen–Tawil syndrome patients as well.21 The mean time to reach an abnormal decrement in periodic paralysis is approximately 25 minutes but may take the full 50 minutes of the test to appear, or perhaps even longer in hypoKPP-2.21 The type 5 pattern is the typical signature of hypoKPP-1 or occasional hypoKPP-2 patients (Fig. 2-19).19 Neither myotonic discharges nor PEMPs are seen. The short-exercise test is normal and the long-exercise test demonstrates the same slowly developing decremental pattern that is seen in the type 4 pattern.19
Considerable loss of motor units may occur without clinically evident weakness. This is particularly true in slowly progressive disorders where collateral sprouting and reinnervation are at least partially compensatory. In patients with ALS, it has been shown that the CMAP amplitude may not reliably decline until the estimated number of motor units drops below 10% of normal.24 Numerous motor unit number estimation (MUNE) techniques have been developed in an attempt to count the number of viable motor units in a given muscle.25 This has been done with the belief that MUNE would represent a more accurate means to monitor disease course or to detect a response to treatment than measurements of strength. All techniques attempt to estimate motor unit number by estimating the average size of the amplitude generated by a single motor unit, and then dividing this number into the maximal CMAP amplitude for the entire muscle.
MUNE is, in large part, a research technique, with limited application in the daily practice of neuromuscular disease. It can be time consuming and, with some techniques, technically challenging due to unstable neuromuscular transmission that may occur with reinnervation. Unlike standard EDX techniques, which can offer a panoramic perspective on multiple nerves and multiple muscles, MUNE is typically done on one or at most a limited number of nerves in a single setting. In addition, individual techniques have their limitations including the nerve/muscle pairs that are accessible to them.25 MUNE has probably been most frequently used in the ALS population and has been successfully utilized in at least two clinical trials.26–28 Multiple authors have demonstrated that MUNE decline and the size of individual MUPs increase sequentially in patients with ALS, consistent with our understanding of the denervating/reinnervating process.24,27–32
Needle EMG is performed by inserting a recording electrode into muscle and assessing the electrical waveforms both at rest and with voluntary muscle activation. EMG is interpreted by determining the type of abnormality within a specific muscle, identifying the pattern of muscles in which those abnormalities occur, and then correlating these results with those of NCS as well as the clinical context. Muscles are typically evaluated under three conditions: at rest assessing insertional and spontaneous activity, with minimal voluntary activation assessing motor unit action potential (MUAP) morphology and stability, and with a gradual increase in muscle activation assessing MUAP recruitment.
Spontaneous activity and insertional activity are similar but not synonymous concepts. Insertional activity refers to the immediate response to needle movement. A brief burst of insertional activity occurring with each needle movement within viable muscle is the means by which the examiner is certain that they are in muscle. Increased insertion can refer to a protracted and nonspecific response to needle movement seen immediately after axon loss as a harbinger of the abnormal spontaneous activity in the form of positive waves and fibrillation potentials in muscles tested early after nerve injury. A number of the forms of abnormal spontaneous activity such as complex repetitive and myotonic discharges are often triggered by needle insertion. In disease, and in numerous other situations, increased insertional and abnormal spontaneous activities occur together.
Spontaneous activity refers to activity that occurs independently of needle movement. It may occur with or without a provoking needle movement but is sustained long after any provoking stimulus ceases. To further emphasize the subtle, but meaningful differences in the terminology, it is possible to have a situation in which there is increased spontaneous activity in the setting of decreased insertional activity, a concept that at first glance may seem counterintuitive. For example, it is not uncommon to experience this scenario while studying intrinsic foot muscles in patients with chronic polyneuropathies. There is often reduced insertional activity, presumably due to replacement of viable muscle by connective tissue. By the same token, there are isolated areas in that muscle where fibrillation potentials can be found, presumably representing isolated, viable, and nonreinnervated muscle fibers.
Spontaneous activity can be normal. End-plate spikes and end-plate noise represent miniature EPPs and are seen in normal muscles at rest when the needle is in proximity to the motor endplate. In contrast, there are a number of abnormal waveforms that may occur in an abnormal muscle at rest. These include fibrillation potentials and positive sharp waves, cramp potentials, myokymic discharges, myotonic discharges, complex repetitive discharges, and neuromyotonic discharges (Figs. 2-20,2-21,2-22,2-23,2-24). Of these, fibrillation potentials and positive sharp waves are the most prevalent (Fig. 2-20). They are recognized by their metronomic and regular firing pattern. When present, these suggest that anatomic continuity has been lost between a muscle fiber and its innervating axon. Less commonly, they may result from myopathies associated with muscle membrane instability. Fibrillation potentials and positive waves occur most commonly from denervation in association with axon loss at any location from anterior horn cell to terminal twig (Table 2-2).33 They do not occur in demyelinating neuropathies in the absence of axon loss.
Site | Type | Examples |
---|---|---|
Anterior horn | Axon loss | ALS, SMA, poliomyelitis, syringomyelia, spinal cord infarction |
Ventral root | Axon loss | Spinal stenosis, neoplastic meningitis |
Plexus | Axon loss | Trauma, tumor, brachial plexus neuritis, diabetic radiculoplexopathy |
Peripheral Nerve | Axon loss | Toxic, metabolic, hereditary, entrapment, and compression |
NMJ | Pre-synaptic | Botulism, LEMSa |
Post-synaptic | Autoimmune myasthenia gravis | |
Muscle | Inflammatory | Dermatomyositis, polymyositis, inclusion body myositis |
Infiltrative | Amyloidosis, sarcoidosis | |
Dystrophic | Dystrophinopathy, limb girdle, myotonic dystrophy | |
Toxic | Cholesterol lowering agents, chloroquine | |
Necrotizing | Anti-signal recognizing protein | |
Inherited metabolic | α glucosidase deficiency | |
Congenital myopathies (certain) | Myotubular myopathy, late onset Nemaline | |
Infectious | HIV, other viral, trichinosis |
Figure 2-20.
Fibrillation potentials and positive waves—single action potentials that usually fire with metronomic frequency; fibrillation potentials have short duration, positive waves with characteristic configuration and longer duration. The sound produced by fibrillations can be described as a “ticking.” Positive waves have a more nonspecific, duller sound than fibrillation potentials, with recognition based more on their firing pattern and characteristic shape. These waveforms may occur in isolation or concurrently as in this figure.

Fibrillation potentials and positive waves are not however, specific for axon loss Table 2-2. They may be observed in both DNMT and certain muscle diseases. In the former, the axon may be effectively separated from its target muscle by ablation of the neuromuscular junction. In myopathy, particularly those associated with the pathologic features of segmental necrosis of muscle or fiber splitting, viable segments of muscle may be separated from segment of muscle containing the neuromuscular junction. By doing so, the myopathic process has effectively denervated segments of viable muscle. There are a number of myopathies, notably the channelopathies, and some of the congenital and mitochondrial myopathies, where these forms of abnormal spontaneous activity occur without clear explanation of the denervating mechanism. Presumably, muscle membrane instability provides an alternative explanation for this form of waveform generation.
Other forms of abnormal spontaneous activity are less common. As some of these discharges have clinical concomitants that go by the same name, this text will follow the convention used by others. The clinical observation will be referred to by the name alone, for example, fasciculation whereas the waveform will be referred to as a potential or discharge, for example, fasciculation potential. Complex repetitive discharges are nonspecific and occur in both chronic nerve and muscle disorders (Fig. 2-22). Although usually considered an indication of nerve or muscle pathology, current thinking suggests that they may be occasionally identified as a normal finding in both the biceps and more commonly, the iliopsoas muscles. Complex repetitive discharges have a machinery-like sound that typically starts and stops abruptly. Whether related to nerve or muscle disease, they are thought to originate from a reverberating circuit that develops within contiguous myofibers. Complex repetitive discharges are typically unassociated with any observable clinical concomitant.
Myotonic discharges also appear to originate from muscle and are primarily associated with heritable muscle disease (Fig. 2-23). These produce a waxing and waning sound historically likened to a dive bomber. From a more contemporary perspective, a revving chain saw or motor bike may represent a more apt simile. Myotonic discharges do not sustain themselves and typically dissipate until such time that they are provoked by the next needle movement or muscle contraction. Their presence often obscures the ability to adequately study MUAPs or assess other forms of abnormal spontaneous activity. Myotonic discharges are the major EDX signature of myotonic muscular dystrophies, (particularly DM1) the nondystrophic myotonias associated with chloride and certain sodium channel disorders, and in some patients with hyperKPP.33,34 These are also seen in certain glycogen storage disorders, most notably acid maltase deficiency, branching and debranching enzyme deficiencies, myofibrillar myopathy, and occasionally in toxic and inflammatory myopathies (Table 2-3). Myotonic discharges are not clinically observable but are commonly associated with muscle stiffness.
Myotonic muscular dystrophy types 1 and 2 Myotonia congenita Paramyotonia congenita Other nondystrophic myotonias, e.g., potassium-aggravated myotonia Hyperkalemic periodic paralysis Azacholesterol Monocarboxylic acids Colchicine myopathy Cholesterol lowering agent myopathy Hypothyroidism Inflammatory myopathies (rare) Acid maltase deficiency Branching enzyme deficiency myopathy Debranching enzyme deficiency myopathy Hereditary vacuolar myopathies (Danon disease and X-linked myopathy with excessive autophagy) Myofibrillar myopathy and other distal myopathies with rimmed vacuoles Welander myopathy |
Other forms of abnormal spontaneous activity appear to be generated by nerve such as fasciculations potentials, myokymic and neuromyotonic discharges. All of them can be conceptualized as part of nerve hyperexcitability spectrum disorders.15 Fasciculation potentials are singular, spontaneous discharges of single MUPs that represent the electrophysiologic correlate of the fasciculations that can often be seen while observing the muscle with the naked eye. They can be seemingly benign (or at least associated with subclinical pathology) or associated with any one of a number of nerve diseases. They are most closely linked and probably most commonly seen in anterior horn cell diseases. Numerous authors have attempted to identify the morphologic or behavioral characteristics of fasciculation potentials that would distinguish benign from more ominous forms. There have been recent publications that promote the concept that complex and unstable fasciculation potentials correlate with pathology and can be used as a surrogate marker for fibrillation potentials and positive waves in the electrodiagnostic evaluation of ALS patients.35–37 Ironically, a contributing author to one of these papers has studied fasciculation potentials and has concluded that there are no characteristics that confidently allow the distinction of benign from pathologic fasciculations.38 The ability to observe clinical fasciculations, in association with fasciculation potentials, is undoubtedly related to the thickness of subcutaneous tissue, the depth of the muscle, and whether the fasciculations arise from superficially placed motor units within that muscle.
Cramp potentials are groups of spontaneously firing, otherwise normal MUPs that discharge in a sputtering pattern, typically with abrupt onset and cessation. Like fasciculations, they are typically neurogenic in origin and may have either a benign or pathologic significance. They are commonly found in chronic neuropathic conditions such as motor neuron diseases, radiculopathy, or polyneuropathy but can occur in any of the nerve hyperexcitability syndromes discussed subsequently in the following paragraphs on myokymic and neuromyotonic discharges or in a number of metabolic disturbances including pregnancy, uremia, and hypothyroidism.33 Cramp potentials are typically associated with clinical cramping.
Myokymic discharges are grouped discharges of two or more MUPs that fire repeatedly in a semirhythmic manner with interval gaps of electrical silence. The intraburst frequency is between 40 and 150 Hz. The sound produced has been likened to troops marching. These probably result from ephaptic transmission between injured, contiguous axons. Myokymic discharges can be seen in a number of different etiologies of nerve disease (Fig. 2-21 and Table 2-4). These may be associated with disorders as mundane as CTS or as unusual as rattlesnake envenomation. In the cranial musculature, these are most commonly associated with multiple sclerosis, GBS, and brainstem neoplasms. These are common in disorders of neural overactivity such as the cramp fasciculation syndrome and Isaac’s syndrome. Myokymic discharges are most commonly associated with radiation-induced nerve injury. Myokymic discharges may or may not be associated with the recognition of clinical myokymia.
Location | Disorder |
---|---|
Facial muscles | Multiple sclerosis |
Brainstem glioma | |
Guillain–Barré syndrome | |
Bells palsy | |
Head and neck radiation | |
Limb muscles | Radiation |
Chronic nerve compression, e.g., CTS | |
Isaac’s syndrome and its differential diagnosis (see Table 2-5) |
Neuromyotonic discharges are infrequently seen (Fig. 2-24). They are commonly associated with other waveforms such as myokymic discharges, cramp discharges, and fasciculation potentials that occur in disorders of neural hyperexcitability. Their duration is brief as their high frequency (150–300 Hz) precludes them from sustaining themselves for protracted periods. Their sound has been likened to the scream of a formula-one race car engine. These are most closely linked to the disorder known by the names neuromyotonia, Isaac’s syndrome, or the syndrome of continuous muscle fiber activity. Neuromyotonic discharges are nonspecific and may be associated with a number of neurogenic disorders, some of which are paraneoplastic (Table 2-5).21,33,39 Although initially some of these cases were attributed to antibodies directed against voltage-gated potassium channels(VGKCs), subsequent studies have revealed that these antibodies actually are directed against Caspr2.17 An analogous discharge, the neurotonic discharge, may be recorded from muscles during intraoperative monitoring, particularly with acoustic nerve surgery, as a means of detecting potential injury to the facial nerve.
Acquired | Isaac’s or Morvan syndrome |
Cramp fasciculation syndrome | |
Paraneoplastic (thymoma, thyroid carcinoma) | |
Multifocal motor neuropathy | |
IgM kappa paraproteinemic neuropathy | |
Diabetic neuropathy | |
Copper deficiency | |
Creutzfeld–Jakob disease | |
Intraoperative nerve irritation | |
Anticholinesterase poisoning | |
Timber rattlesnake envenomation | |
Radiation | |
Hereditary | Charcot–Marie–Tooth disease |
ALS | |
Distal spinal muscular atrophy | |
Kennedy disease | |
Paramyotonia congenita |
The purpose of examining the muscle with minimal levels of voluntary activation is to assess the morphology and stability of individual MUAPs. The motor unit consists of an anterior horn cell, its axon, and all of the muscle fibers it innervates. The MUAP refers to the collection of all single fiber action potentials, arising from the muscle fibers of that MUP, that are within the recording radius of the needle. MUAPs become smaller when there is a loss or physiologic nonparticipation of muscle fibers within the motor unit. This typically occurs in disorders of muscle and neuromuscular transmission. In the former, the number of fibers within the motor unit decreases as a result of myofiber degeneration. Alternatively, the size of the action potential that each fiber generates decreases as a result of myofiber atrophy. In DNMT, the amplitude and/or duration of the MUAP may decrease from blockade of neuromuscular transmission, physiologically reducing the number of single fiber muscle action potentials (SFMAPs) contributing to that MUAP.
Conversely, MUAPs typically become larger following chronic denervation and reinnervation typically occurring between 3 and 6 months after disease onset.33 An orphaned muscle fiber deprived of its axon supply may be reinnervated by a collateral nerve twig belonging to a neighboring viable motor unit. As a result, surviving motor units in the aftermath of axon loss will typically grow both in amplitude and in duration. Normal values for both amplitude and duration are available but vary depending on patient age and the muscle studied. In general, most MUAPs in most limb muscles are between 8 and 15 μs in duration and less than 2 mV in amplitude. MUAP morphology does not change in a substantive manner in a purely demyelinating nerve disorder, although both increased duration and polyphasia may result from variable conduction slowing in terminal nerve twigs.
MUAPs typically have triphasic configurations, although occasional MUAPs with a few extra turns or phases are common. Polyphasic or serrated MUAP morphology implies that the individual SFMAP components of that MUP have become desynchronized. Satellite potentials, that is, waveforms that are separate from but time-locked to the primary MUAP waveform, are considered as a subtype of polyphasia. An abundance of polyphasic MUAPs implies that the process, if monophasic is subacute, that is, of weeks’ to months’ duration.33 Polyphasic MUAPs have no etiologic specificity and can be seen in myopathies, axon loss, and demyelinating nerve disease. Polyphasic motor units will eventually reconfigure themselves into a triphasic configuration, even in those MUPs that have been exceptionally enlarged in remote denervating and reinnervating disease such as polio.
The concepts outlined in the preceding three paragraphs are generally accurate but have exceptions. Large motor units reminiscent of reinnervation following denervation occur in chronic myopathies, particularly inclusion body myositis.33,41,42 Long-duration MUAPs in myopathy have been hypothetically attributed to three different mechanisms all of which are related to regenerating fibers. The nerve fibers that innervate regenerating muscle fibers may have slow conduction velocities relating to their immaturity. Regenerating muscle fibers may have neuromuscular junctions scattered along their length rather than condensed near a single motor point. Either of these may lead to delayed arrival of the muscle fiber action potential between the site of its origin and the site of the recording electrode, thus dispersing the SFMAP components within the MUP. Lastly, the conduction velocity of the regenerating muscle fiber itself may have this same dispersal effect.42
Conversely, in certain axon loss disorders, small motor units referred to as nascent units may be seen. These occur because of a concomitant reduction in MUP size and MUP number. A severe monophasic nerve injury with reinnervation that allows for only a limited number of myofibers to be initially incorporated into the motor unit is probably the most common situation in which nascent units occur. These may also be seen in rapidly evolving denervating disorders in which effective reinnervation does not occur, and in which degeneration of terminal twigs occurs early in the disease course. The familial form of ALS associated with the A4 V mutation of the SOD1 gene is perhaps the most notable example of this latter mechanism. In either case, small motor units from nerve and muscle diseases are most readily distinguished from one another by their characteristic decreased and increased recruitment patterns respectively as described below.
MUP variability or instability refers to the alteration of MUP morphology on consecutive discharges (Fig. 2-25). Under normal circumstances, every single fiber action potential within a given motor unit discharge in a near concordant fashion. As a result, as long as the needle position is stable, the MUAP will have an identical configuration each time it discharges. With disordered neuromuscular transmission of any cause, transmission at one or more individual neuromuscular junctions may be delayed or actually fail. As this is a physiologic rather than a direct structural effect, this provides a dynamic process affecting different neuromuscular junctions variably with consecutive discharges. As a result, the configuration of the MUP may change quite dramatically with consecutive discharges. Variability of amplitude is best demonstrated by having the patient minimally activate the muscle being tested, in order to isolate an individual MUP while using slow sweep speeds (50–100 ms/division) and high settings on low-frequency filters, similar to what is deployed in SFEMG. This allows the examiner to visualize the same MUP multiple times on the same screen. MUP variability is most closely associated with DNMT. It can often be seen however, in early reinnervation where neuromuscular junctions are immature. Traumatic nerve injury and ALS are notable examples.
As the last component of the needle examination, the examiner attempts to estimate the number of MUAPs that the patient is capable (or willing) to activate. This is done by having the patient gradually increase the amount of isometric resistance in the muscle being tested. Under normal circumstances, an increasing number of MUPs will be recruited, that is, seen and heard on the EMG machine display. Each has its own distinctive sound and configuration as the makeup and topographical relationship of each MUAP to the recording electrode differs with any needle location.
In axon loss, and in demyelinating neuropathies with conduction block, there are a reduced number MUAPs that can be recruited. As a result, the remaining activatable MUAPs that fire do so with a greater frequency than normal in an attempt to compensate for MUAP loss. This is referred to as reduced recruitment. Reduced recruitment is one cause of a reduced interference pattern. Again, these are two terms that are related, but not synonymous. A reduced interference pattern may also occur as a result of a patient’s inability or unwillingness to fully activate all MUAPs at their disposal due to disorders of the central nervous system, pain, or malingering. In this case, there will be no rapidly firing MUAPs identified despite a reduced number of MUAPs on the screen as there is no stimulus to do so.
Many strategies are utilized in an attempt to quantitate MUAP loss. More often than not, reduced recruitment is estimated by training one’s ear to detect MUAPs firing at rates that are excessive. The ear is quite sensitive in its ability to detect rapidly firing MUAPs in response to motor unit loss. Many electromyographers can accurately estimate the firing frequency of an MUAP to within 2 Hz, certainly within 5 Hz. With full recruitment resulting in a full interference pattern, there will be an amorphous blending of the sound created by all activated motor units. With reduced recruitment, rapidly firing individual MUAPs can be detected by hearing the distinctive sound that each MUAP firing at an accelerated pace produces. This effect has been likened to the sound produced by a baseball card placed in the spokes of a child’s moving bicycle wheel. This can be done at very low levels of recruitment by estimating the firing frequency of the first MUAP when the second MUAP is recruited, and the firing frequency of the second MUAP when the third MUAP is recruited and so on. Typically, firing frequency of an MUAP at onset is 6–8 Hz. The second MUAP is typically recruited by the time this MUAP is firing at 10 or 12 Hz. If a single MUP is firing at a rate greater than 12 Hz (in limb muscles) and no other MUAP is recruited, this is an objective measure of decreased recruitment. Hearing MUAP firing faster than this is an accurate indicator of axon loss or demyelinating conduction block. This is the method espoused by Daube and Rubin and represents the preferred method by the authors because of its combined accuracy and efficiency.33
There are more objective methods that can be utilized by someone who has not yet developed a trained ear. The recruitment ratio is an alternative method This involves identifying the rate of the fastest firing MUP and dividing it by the number of MUAPs that have been activated. This number should never exceed 5 in normal circumstances. For example, if the most rapidly firing of two recruited MUAPS were discharging at a rate of 15 Hz, and only two MUPs were activated, this ratio of 7–1/2 would represent reduced recruitment.
In myopathies or DNMT, the problem is not a reduced number of motor units but reduced MUAP size. As the amount of force generated by individual MUAPs is often reduced, more motor units need to be recruited earlier than normal to generate the same amount of force. The firing rates of these MUAPs remain normal. This effect has been termed as early or increased recruitment. In general, increased recruitment, proportionate to effort rendered, is a subjective and fairly insensitive EDX measure and is usually not recognized until other EDX features of muscle disease or DNMT are evident.
SFEMG has a number of potential applications. Its primary clinical application is to enhance the sensitivity of DNMT testing. Unfortunately, what is gained in sensitivity is often lost in specificity. For this reason, its greatest utility lies in its ability to discriminate between subtle presentations of DNMT from nonneuromuscular diseases. It is limited in its ability to distinguish one neuromuscular disorder from another. In general however, large jitter values associated with frequent neuromuscular blocking is more likely to represent a DNMT than in diseases of the anterior horn cell, nerve, or muscle.
The basic goal of SFEMG is to capture and analyze two or more SFMAPs belonging to the same MUAP. In order to do so, it is necessary to limit MUAP recruitment to be able to see, hear, and cleanly record these SFMAPs. In cooperative patients, this can be accomplished on a voluntary basis. In patients who are unable to activate a limited number of MUAPs and maintain a stable level of recruitment for any reason, stimulated SFEMG, as described below, may well represent a better option. Historically, there are two significant technical differences between SFEMG and standard EMG recordings. Both involve limiting the recording radius of the needle so as to facilitate SFMAP acquisition and analysis. Limiting the recording radius involves increasing the low-frequency (high-pass) filter setting to 500 Hz. The second means to limit the recording radius is to use a special SFEMG needle whose recording radius is smaller than its concentric or monopolar counterparts. In part due to the cost and inconvenience involved in the use of SFEMG needles, that require both repeated sterilization and sharpening, electromyographers are now utilizing standard, disposable concentric EMG needles. Normative jitter data for concentric needles are approximately 5 μs less at any given age for any given muscle, in comparison to published norms using SFEMG needles.221
The primary parameter measured by SFEMG is jitter which represents the variation in the interval between two SFMAPs belonging to the same MUAP. Jitter is a property of normal neuromuscular transmission. Its physiologic basis is derived from the variable nature of the EPP as was described in the section on repetitive nerve stimulation. The key to understanding the origin of jitter is the knowledge that the slope of the rise in membrane potential that occurs between the resting membrane potential and action potential threshold is proportionate to the amplitude of the EPP. In other words, the higher the EPP, the steeper the slope and the shorter the interval between the inciting nerve and the resulting muscle fiber action potential. As a result, the interval between consecutive discharges of two SFMAPs belonging to the same motor unit will fluctuate with the normal variation in quantal ACH release and resultant EPP amplitude and slope.
In practice, two (or more) SFMAPS are captured by using a triggered delay line. To do so, the electrodiagnostician will carefully manipulate the EMG needle until it is within an acceptable recording distance from at least one SFMAP. The optimal needle position is determined by listening for generation of the sharp, crisp sound that is generated by this proximity and then confirming the proximity by assuring that the rise time of the waveform is less than 300 μs with an amplitude that is greater than 200 μV. Typically, this is done with a sweep speed of 1 ms/division and a gain of 100–200 μV/division.
Once this SFMAP is isolated, subtle movements of the electrode are made in an attempt to find a second SFMAP belonging to the same motor unit, identified by its “time-locked” characteristics. The interval between the two (or more) spikes should be far enough apart to be measurable but not farther than 4 ms, for purposes of measurement accuracy. Ideally, a steady, low level of contraction will allow the recording of a 100 consecutive discharges of this fiber pair. At least 50 of these 100 discharges must be captured to allow for accurate statistical analysis. Under normal circumstances, the waveforms representing the second of the two SFMAP waveforms will be nearly overlapping with each other but will not be perfectly superimposed. This variation between the first and fluctuating second single muscle fiber action potentials is the basis of normal jitter. (Fig. 2-26). This variation in interpotential interval is usually expressed as the mean consecutive difference (MCD), a calculation readily computed by most contemporary EMG machines. The mean sorted difference (MSD) is also calculated and is a more accurate measure of jitter if the MCD/MSD ratio exceeds 1.25.
Figure 2-26.
Single fiber electromyography demonstrating a normal recording (A) and increased jitter and blocking (seventh pair from top—arrow) (B).
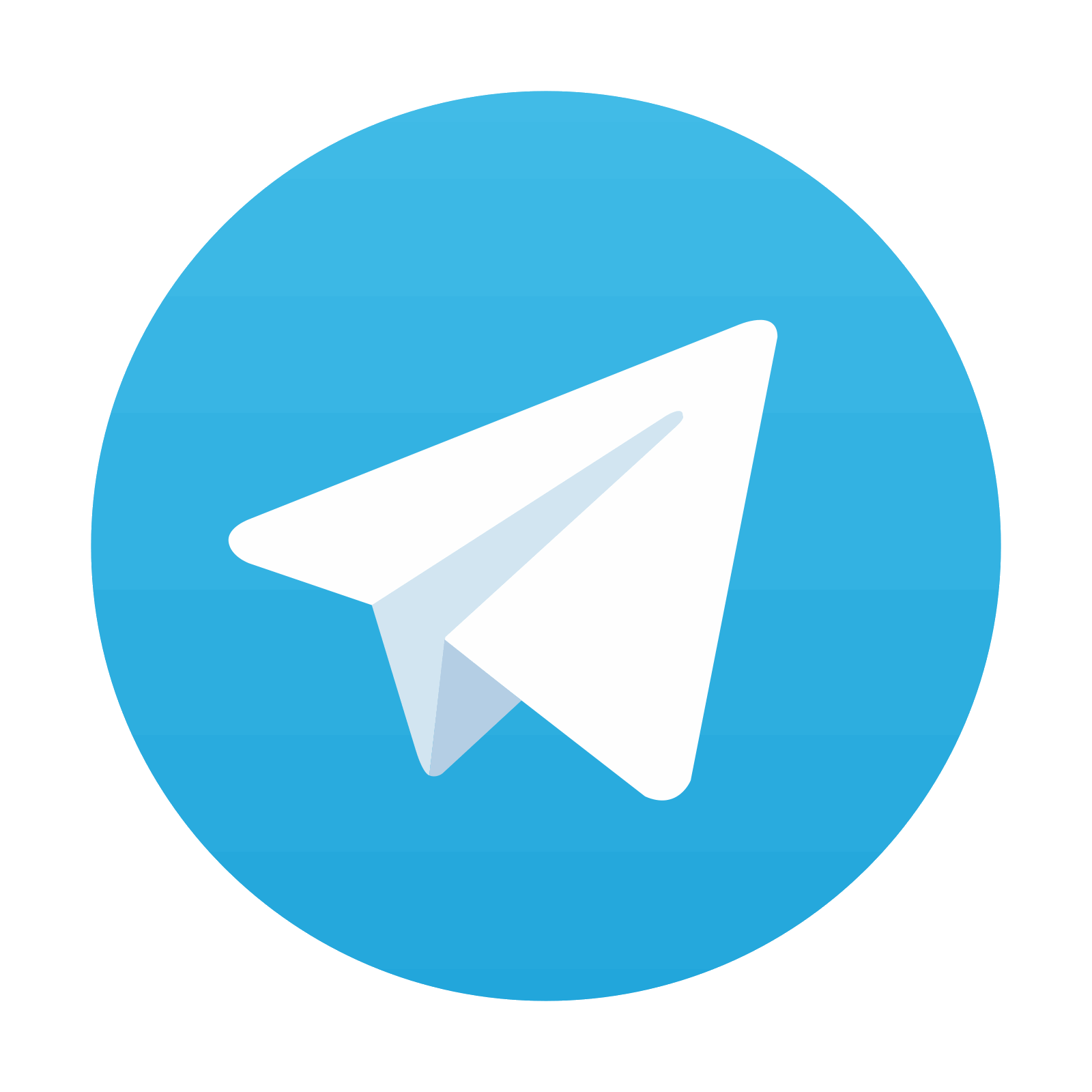
Stay updated, free articles. Join our Telegram channel
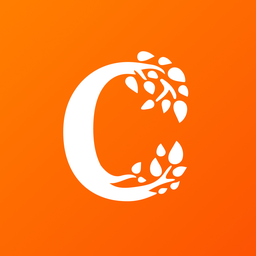
Full access? Get Clinical Tree
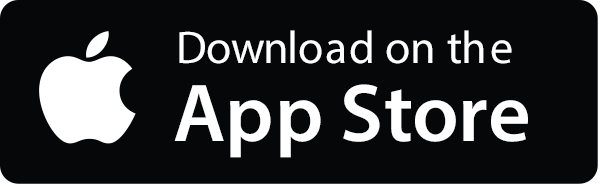
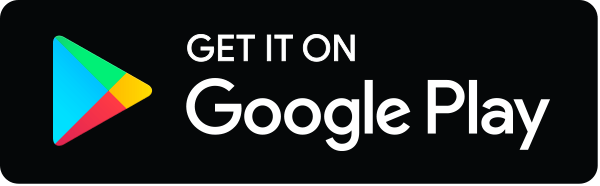
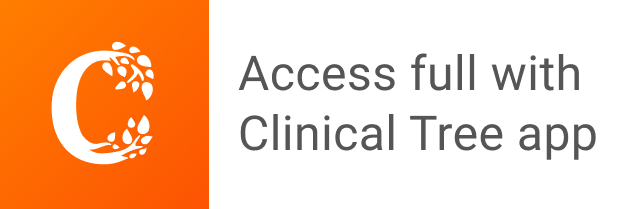