Thalamocortical Anatomy and Physiology
Hal Blumenfeld
Douglas A. Coulter
Introduction
The thalamus and the thalamocortical system participate intimately in the generation of generalized seizures and probably are involved in the synchronization and propagation of localization-related seizures as well.11,13,14,108 This is due to a combination of factors that will be discussed in this chapter, including chiefly the intimate reciprocal excitatory synaptic relationship between the thalamus and cerebral cortex, and the intrinsic properties of the thalamus and associated structures that predisposes the system to generate synchronized rhythms. We will emphasize the role of the thalamocortical system in generating the spike-and-wave discharges (SWDs) seen in generalized seizures, since most work has been done with this seizure type. Continued investigations may allow similar concepts to be applied in localized epilepsy as well.
The importance of the thalamus in generation of seizures has been a subject of active experimentation for at least the past 60 years. In the 1940s, the fact that generalized spike-and-wave seizure discharges appeared virtually simultaneously throughout large areas of neocortex led investigators of the time to hypothesize that there was a “centrencephalic” pacemaker structure synchronizing and driving these rhythms.112,114 Studies by Jasper and colleagues65 and by Dempsey and Morison39 demonstrated that low-frequency stimulation to midline thalamic structures elicited electroencephalographic responses in cortex very similar to generalized SWDs of generalized absence epilepsy. Depth recordings in patients established that the SWDs of generalized absence epilepsy were generated by an underlying thalamocortical oscillation.5,13,52,106,113,122,126,146,147 Detailed studies of anatomic and physiologic mechanisms underlying generation of normal rhythms in the thalamocortical system have clearly delineated the underlying structures critical to the generation of synchronized rhythms in this system: the thalamic relay nuclei, the surrounding γ-aminobutyric acid (GABA)ergic nucleus reticularis, and the neocortex. Recent work suggests that epileptic activity and altered excitability occurs in selective regions of the thalamocortical network and spares others. This selective involvement may provide more specific targets for therapeutic interventions.
Anatomy of the Thalamus
Gross Structure
The thalamus can be subdivided, based on its gross anatomy, into three main nuclear masses: anterior, medial, and lateral. They are separated by a white-matter tract called the internal medullary lamina. In addition, a fourth group consists of several small nuclei found within the internal medullary lamina, termed the intralaminar nuclei, and extends to the midline, forming the midline thalamic nuclei. These four groups of nuclear masses share a common embryologic origin and have collectively been termed the dorsal thalamus. An important functional partner of the thalamus is the nucleus reticularis thalami (NRT), which in coronal sections is a shell-shaped, thin layer of cells surrounding the thalamus on its lateral and rostral extent—separated from the thalamus proper along most of its extent by the external medullary lamina. The NRT has a distinct embryologic origin from the remainder of the thalamus and has been grouped within the ventral thalamus (also comprised of the ventral lateral geniculate nucleus and zona incerta), which, unlike the thalamus proper, does not send axonal fibers to the cerebral cortex. Within each nuclear group in the dorsal thalamus, it is possible to distinguish subnuclei based on their appearance in Nissl-stained sections.
Different Nuclei
Thalamic nuclei can also be classified based on their main input and output connections (Table 1). Thalamocortical cells in the thalamic relay nuclei convey nearly all information en route to the cerebral cortex. Projections from some relay nuclei to thalamus are localized to specific cortical regions, whereas projections from other nuclei are more diffuse (Table 1). In addition, the relay nuclei receive massive reciprocal connections returning from the cortical areas to which they project. The intralaminar nuclei are distinguished by their major reciprocal connections with the basal ganglia, in addition to widespread reciprocal connections with the cerebral cortex. The NRT is unique in that it receives inputs, but sends no outputs to the cerebral cortex, and also has reciprocal connections with all thalamic nuclei.
Thalamocortical Circuits and Other External Connections
The basic synaptic circuit comprising the thalamocortical system is repeated with only modest modifications in most of the primary sensory relay nuclei. This simplified functional circuit will be employed to develop the concept and discuss the rhythm-generating functions of the thalamocortical system, the main focus of this chapter. This basic synaptic circuit is discussed in the next section and schematically depicted in FIGURE 1.
Reciprocal Connections Between Thalamus and Cortex
The principal sensory relay nuclei within the thalamus send an ordered projection to layers III and IV and to the V–VI border of cortex (synapse 1 and 2, Fig. 1) and receive a feedback
projection from the layer VI pyramidal neurons of the same area of cortex (synapse 3, Fig. 1). Both the thalamocortical and corticothalamic projections also send axon collaterals to the surrounding NRT (synapses 4 and 5), which, in turn, provides inhibitory feedback onto the thalamus (synapse 6, Fig. 1). Both the corticothalamic and thalamocortical projections are excitatory and use glutamate or aspartate as a neurotransmitter, whereas NRT uses GABA as a neurotransmitter (Fig. 1).
projection from the layer VI pyramidal neurons of the same area of cortex (synapse 3, Fig. 1). Both the thalamocortical and corticothalamic projections also send axon collaterals to the surrounding NRT (synapses 4 and 5), which, in turn, provides inhibitory feedback onto the thalamus (synapse 6, Fig. 1). Both the corticothalamic and thalamocortical projections are excitatory and use glutamate or aspartate as a neurotransmitter, whereas NRT uses GABA as a neurotransmitter (Fig. 1).
Table 1 Major Thalamic Nuclei | ||||||||||||||||||||||||||||||||||||||||||||||||||||||||||||||||||||||||||||||||||||||||||||||||||||||||||||||
---|---|---|---|---|---|---|---|---|---|---|---|---|---|---|---|---|---|---|---|---|---|---|---|---|---|---|---|---|---|---|---|---|---|---|---|---|---|---|---|---|---|---|---|---|---|---|---|---|---|---|---|---|---|---|---|---|---|---|---|---|---|---|---|---|---|---|---|---|---|---|---|---|---|---|---|---|---|---|---|---|---|---|---|---|---|---|---|---|---|---|---|---|---|---|---|---|---|---|---|---|---|---|---|---|---|---|---|---|---|---|
|
In contrast, the midline (intralaminar) thalamic nuclei also send projections to cortex (with a collateral projection to NRT), but this projection is much more diffuse than is the ordered projection from sensory relay nuclei, and terminates in superficial and deep layers (I and VI). These intralaminar nuclear projections are concentrated in the frontal, medial, and dorsolateral cortex, but intralaminar projections may reach all cortical areas.56 As with the sensory relay nuclei, the intralaminar nuclei receive feedback projections from the cortex but, unlike the corticothalamic feedback projections onto principal sensory relay nuclei, intralaminar nuclei receive this projection from smaller layer V pyramidal neurons of cortex. In addition, the intralaminar nuclei project to the neostriatum and receive a feedback projection from the globus pallidus, as well as from the NRT.
Local Intrathalamic Circuits
Essentially, there are no local intrathalamic circuits involving interconnections of thalamocortical relay neurons within or between thalamic nuclei. The principal axonal target of these neurons is the ipsilateral cerebral cortex, and this projection was discussed earlier. However, there are significant populations of local circuit neurons within thalamic nuclei, and these neurons usually are GABAergic. In addition, NRT provides an extensive and powerful feedforward and feedback inhibitory projection onto relay neurons of the dorsal thalamus, and this intrathalamic inhibitory connection is of fundamental importance in the rhythm-generation capabilities of the thalamocortical system.
GABAergic local circuit neurons modulate the strength of afferent input into the thalamus. These neurons participate in a distinct form of synaptic arrangement in which dendrites of interneurons act as both pre- and postsynaptic elements in a large, glial-ensheathed synaptic aggregation that also contains the large afferent sensory terminal, dendritic appendages from relay neurons, and conventional inhibitory terminals (synapses 8–10, Fig. 1). The interneuron dendrite receives excitatory synaptic input from the sensory afferent terminal (synapse 9, Fig. 1), which also provides excitatory input onto the
neighboring thalamic relay neuron dendrite (synapse 8, Fig. 1). The interneuron dendrite also forms an inhibitory synaptic connection with the thalamic relay neuron dendrite (synapse 10, Fig. 1).125 This synaptic arrangement has been termed a synaptic triad (Fig. 1). One of the primary functions of these synaptic specializations within the thalamus is to dramatically shorten the duration of afferent excitation of thalamic relay neurons through rapid truncation of the sensory excitatory postsynaptic potential (EPSP) before it can reach the thalamic relay neuron soma and there be transduced into action potential output to the cortex. This may serve a role in more the faithful temporal relay of sensory information to the cerebral cortex.
neighboring thalamic relay neuron dendrite (synapse 8, Fig. 1). The interneuron dendrite also forms an inhibitory synaptic connection with the thalamic relay neuron dendrite (synapse 10, Fig. 1).125 This synaptic arrangement has been termed a synaptic triad (Fig. 1). One of the primary functions of these synaptic specializations within the thalamus is to dramatically shorten the duration of afferent excitation of thalamic relay neurons through rapid truncation of the sensory excitatory postsynaptic potential (EPSP) before it can reach the thalamic relay neuron soma and there be transduced into action potential output to the cortex. This may serve a role in more the faithful temporal relay of sensory information to the cerebral cortex.
Brainstem Neuromodulatory Afferents
In addition to the excitatory sensory afferents that innervate the dorsal thalamic relay nuclei, other inputs reach the thalamus and cerebral cortex from various brainstem sources. These afferents release a number of different neurotransmitters. Cholinergic afferents arrive from the basal forebrain, pedunculopontine tegmental and lateral tegmental nucleus; noradrenergic afferents arrive from locus ceruleus; serotonergic afferents originate from the dorsal raphe; and histaminergic afferents originate from the tuberomammillary nucleus of the hypothalamus. Activation of these neuromodulatory afferents serves principally to maintain the thalamus in a “relay” mode (i.e., where afferent sensory activity is faithfully relayed to the cortex). Reduction in the activity in these afferents results, in general, in an enhancement of low-frequency oscillatory activity in the thalamocortical system.90
Physiology of Thalamic Neurons
Neurons in virtually all areas of the thalamus are characterized physiologically by the presence of a large-amplitude, low-threshold calcium spike.63,64 This intrinsic membrane conductance underlies bursts of action potentials generated in thalamic neurons and plays an important amplifying role in thalamic oscillatory behavior, including sleep spindles and the spike-and-wave discharges (SWDs) of generalized absence epilepsy. In contrast to its size in other mammalian neurons, the low-threshold calcium (Ca) current is particularly prominent in thalamic neurons, where its overall peak amplitude is usually equal to or greater in amplitude than the high-threshold (dihydropyridine-sensitive) calcium current in the same cells.30 This proportionally large low-threshold (“T”-type) Ca current influences the behavior of thalamic neurons and, in situations in which thalamic neurons are hyperpolarized from their normal waking resting membrane potentials, dominates the cellular properties of these neurons.63 The anomalous dependence on hyperpolarization for activation of low-threshold Ca current is explained by the underlying characteristics of this current. The biophysical properties of the low-threshold Ca current are similar in many ways to those of the classical sodium (Na) current underlying action potential generation. The T current is activated at potentials very close to the resting potential of neurons, with a threshold for activation around -60 mV (Fig. 2A).32 However, the T current is also inactivated by potentials near the normal resting potential of thalamic neurons, with virtually all the T channels inactivated at -60 mV (Fig. 2B).30 For T-type Ca channels to be activated, the neuronal membrane potential must be hyperpolarized below -60 mV for a sufficient time to allow the channels to “deinactivate” or become able to be activated. Once this deinactivation process occurs, a subsequent depolarization will allow these Ca channels to open and generate the regenerative low-threshold Ca spike that is the amplifier underlying and generating oscillatory behavior within the thalamocortical system (Figs. 3 and 4). This deinactivation–activation–inactivation sequence of functional states of the T current underlying low-threshold Ca spikes in thalamic neurons is a fundamental process that is repeated over and over during the generation of low-frequency rhythms within the thalamus and within the thalamocortical system. Neurons within the NRT have a T current with similar voltage dependence and pharmacology but slower kinetics than those seen in thalamic neurons.60 The genes encoding T-type Ca currents have been cloned, and three T-type Ca channels have been characterized: Cav3.1 (α1G), Cav3.2 (α1H), and Cav3.3 (α1H).49,115,116 Cav3.1 is predominantly expressed in thalamic relay neurons, and Cav3.3 in NRT neurons,132 which explains the difference in T-current properties evident in the two populations of neurons.
In addition to the T-type Ca current underlying the low-threshold Ca spike, thalamic neurons are endowed with another variety of intrinsic membrane current that significantly contributes to the propensity of these neurons to generate low-frequency rhythms. This current is a hyperpolarization-activated cationic current, Ih. This current is carried by both Na+ and potassium (K+) ions, and is activated by hyperpolarization to potentials more negative than -60 mV (Fig. 2C). Ih activates slowly and results in generation of a depolarizing pacemaking potential (Fig. 3A, B).92 When the neuron is in a membrane potential range of -70 to -85 mV, this depolarizing pacemaking potential can then activate a low-threshold Ca spike, which will deactivate the h current and, upon repolarization, Ih is reactivated, once again generating a depolarizing pacemaking potential, activating another low-threshold spike, and so on (Fig. 3A, B). The rate at which Ih activates determines the frequency of low-threshold spike firing, and this, in turn, depends on the resting membrane potential of the neuron because the kinetics of Ih are voltage-dependent, with activation occurring more rapidly during greater hyperpolarizations. This combination of the T-type Ca current and the hyperpolarization-activated cationic current Ih will generate spontaneous, low-frequency oscillatory activity in thalamic neurons when in a membrane potential range of -65 to -75 mV, even in the absence of any synaptic input (Fig. 3A, B).78,92 However, for this activity to become synchronized between cells, the synaptic network of which these thalamic neurons are members must play a critical role.
![]() FIGURE 2. Intrinsic membrane properties of thalamic neurons that contribute to their propensity to generate low-frequency rhythms. A,B: Biophysical properties of the low-threshold T-type Ca current. A: Current voltage plot illustrating activation of the T current by depolarizing steps in an acutely isolated rat thalamic neuron. Note that the T current activates at very hyperpolarized potentials (-60 mV), and that the T current inactivates (i.e., is transient). From Coulter DA, Huguenard JR, Prince DA. Differential effects of petit mal anticonvulsants and convulsants on thalamic neurones: calcium current reduction. Br J Pharmacol. 1990;100(4):800–806, with permission.32 B: Inactivation of the T current by increasingly depolarized membrane potentials in a rat thalamic neuron. Note that the T current requires the membrane potential to be hyperpolarized below -60 mV for the current to be able to be activated, and that the T current is half-inactivated at -80 mV. Modified from Coulter DA, Huguenard JR, Prince DA. Calcium currents in rat thalamocortical relay neurones: kinetic properties of the transient, low-threshold current. J Physiol. 1989;414:587–604, with permission.29 C: The hyperpolarization-activated cationic current Ih. Note that increasing hyperpolarizations activate a greater and greater proportion of the h current, which is half-activated at a membrane potential of -75 mV. From McCormick DA, Pape HC. Properties of a hyperpolarization-activated cation current and its role in rhythmic oscillation in thalamic relay neurones. J Physiol. 1990;431:291–318, with permission.93 |
![]() FIGURE 3. Oscillatory and tonic firing modes of thalamic neurons. A, B: The interaction of T current (It) and Ih results in the generation of spontaneous oscillations in thalamic neurons. A, C: Depolarization of thalamic neurons inactivates It, causing a transition from oscillatory firing mode to tonic relay mode. From McCormick DA, Pape HC. Properties of a hyperpolarization-activated cation current and its role in rhythmic oscillation in thalamic relay neurones. J Physiol. 1990;431:291–318, with permission.93 |
Generation of Normal Thalamocortical Rhythms
In the awake animal, thalamic neurons are maintained at a resting potential of -50 to -60 mV by the combined effects of normal afferent activity and by the ascending activating systems of the brainstem (see earlier discussion); the T current is inactivated; and the thalamus serves as a faithful relay of all types of sensory information to the cerebral cortex (Fig. 3, tonic mode). In this state, the thalamus transduces incoming synaptic input into a patterned action potential frequency output to cortex, with little or no loss of information content. Loss of conscious perception associated with drowsiness or sleep is associated with a marked alteration in the functional properties of the thalamus. Thalamic neurons hyperpolarize as a period of drowsiness or sleep begins,70 due to a reduction in the neuromodulatory activity of the ascending activating systems and a decrease in normal afferent activity; the T current deinactivates in thalamic neurons, and the thalamus transitions from a faithful relay mode of firing to an oscillatory or burst mode of firing (Fig. 3, oscillatory mode). Because of this change in the “state” of thalamic neuron firing properties, during drowsiness or sleep, the thalamus serves more to disrupt and filter sensory
information, and generates low-frequency oscillations during this state of reduced consciousness. Deep stages of sleep are associated with several varieties of rhythmic oscillations in the brain that are generated principally in cortical and thalamic synaptic networks. The early stages of slow-wave sleep are characterized by spindle waves recorded in the electroencephalogram (EEG). These spindle waves consist of 11- to 15-Hz oscillations that wax and wane in amplitude and are usually 2 to 4 seconds in duration, reappearing every 3 to 10 seconds. These events are triggered by an oscillatory interaction between the thalamus and NRT, and can occur spontaneously when these structures are isolated from their cortical connections17,29,61,101,142—that is, the neocortex is not required for generation of these events. Intracellular recordings in vivo have clearly shown that NRT neurons are firing in a series of bursts during spindle discharges, superimposed on a slow depolarization.128 Synchronization of NRT may be enhanced by the presence of dendrodendritic,40 electrical,76,82 and conventional axonal reciprocal inhibitory interconnections between NRT neurons (synapse 7, Fig. 1). Unlike the very specific reciprocal excitatory connections between thalamus and cortex, NRT–thalamic inhibitory connections tend to be much more divergent, with extensive NRT axonal arbors in the thalamus. Therefore, these bursts in NRT elicit synchronized GABAergic inhibitory postsynaptic potentials (IPSPs) in large populations of thalamic relay neurons, mediated by activation of both GABAA and GABAB receptors (Fig. 4).17,73 Due to deinactivation and subsequent activation of T current in thalamic neurons by the IPSP-induced hyperpolarization, a large Ca-dependent spike with an associated burst of Na-dependent action potentials is generated on the rebound of the NRT-mediated IPSPs (Figs. 3B, 4B). These thalamic bursts then reactivate the NRT and activate the cerebral cortex. The reactivated NRT then rapidly reinitiates a second wave of the spindle by triggering another IPSP in thalamic neurons. In this spindle rhythm, the NRT may serve as the pacemaker because thalamic neurons do not exhibit spindle rhythms when isolated from the NRT, whereas the NRT apparently will spontaneously trigger spindles when isolated from the thalamus.128,142 The termination of spindle oscillations may depend on progressive intracellular Ca2+ accumulation, which leads to persistent activation of Ih, disrupting the pacemaker potential for this rhythm.8,84,85
information, and generates low-frequency oscillations during this state of reduced consciousness. Deep stages of sleep are associated with several varieties of rhythmic oscillations in the brain that are generated principally in cortical and thalamic synaptic networks. The early stages of slow-wave sleep are characterized by spindle waves recorded in the electroencephalogram (EEG). These spindle waves consist of 11- to 15-Hz oscillations that wax and wane in amplitude and are usually 2 to 4 seconds in duration, reappearing every 3 to 10 seconds. These events are triggered by an oscillatory interaction between the thalamus and NRT, and can occur spontaneously when these structures are isolated from their cortical connections17,29,61,101,142—that is, the neocortex is not required for generation of these events. Intracellular recordings in vivo have clearly shown that NRT neurons are firing in a series of bursts during spindle discharges, superimposed on a slow depolarization.128 Synchronization of NRT may be enhanced by the presence of dendrodendritic,40 electrical,76,82 and conventional axonal reciprocal inhibitory interconnections between NRT neurons (synapse 7, Fig. 1). Unlike the very specific reciprocal excitatory connections between thalamus and cortex, NRT–thalamic inhibitory connections tend to be much more divergent, with extensive NRT axonal arbors in the thalamus. Therefore, these bursts in NRT elicit synchronized GABAergic inhibitory postsynaptic potentials (IPSPs) in large populations of thalamic relay neurons, mediated by activation of both GABAA and GABAB receptors (Fig. 4).17,73 Due to deinactivation and subsequent activation of T current in thalamic neurons by the IPSP-induced hyperpolarization, a large Ca-dependent spike with an associated burst of Na-dependent action potentials is generated on the rebound of the NRT-mediated IPSPs (Figs. 3B, 4B). These thalamic bursts then reactivate the NRT and activate the cerebral cortex. The reactivated NRT then rapidly reinitiates a second wave of the spindle by triggering another IPSP in thalamic neurons. In this spindle rhythm, the NRT may serve as the pacemaker because thalamic neurons do not exhibit spindle rhythms when isolated from the NRT, whereas the NRT apparently will spontaneously trigger spindles when isolated from the thalamus.128,142 The termination of spindle oscillations may depend on progressive intracellular Ca2+ accumulation, which leads to persistent activation of Ih, disrupting the pacemaker potential for this rhythm.8,84,85
Role of Thalamus and Thalamocortical Circuits in Generating Generalized Epileptiform Discharges
Relationship Between Spindles and Spike-and-Wave Discharges
In addition to the generation of normal rhythms like sleep spindles, the thalamocortical system participates in the generation of pathologic rhythms, as well. Perhaps the best characterized of these rhythms is the 3- to 4-Hz SWD activity of absence epilepsy.14 Unlike spindles, which can be recorded in the thalamus of decorticated animals,29,101 intact thalamocortical connections are required for typical SWDs to be generated.47,97 SWDs are bilaterally synchronous thalamocortical oscillations106,122,146 and, as the name implies, consist of repetitive cycles of an early sharp spike associated with action potential firing in thalamus and cortex, followed by a slower wave associated with prolonged inhibition in cerebral cortex118 and probably also in thalamus.127 Several lines of evidence support the concept that similar mechanisms and
synaptic circuitry may underlie the generation of normal sleep spindle rhythms and the pathologic thalamocortical rhythms of generalized absence epilepsy. In patients with generalized absence epilepsy, Kellaway71 has demonstrated that the occurrence of SWDs is remarkably correlated with slow-wave sleep stages. In the feline penicillin-generalized epilepsy model of generalized absence epilepsy, SWDs have been shown to evolve from spindle rhythms following intramuscular injection of penicillin. This transition from 7- to 14-Hz spindle rhythms to 3- to 4-Hz SWDs occurs gradually and is accompanied by the behavioral symptoms of absence.47 Consistent with the hypothesis that the T current amplifies and drives both normal and pathologic thalamocortical oscillations is the finding that specific generalized absence anticonvulsants like ethosuximide (Zarontin) and trimethadione (Tridione) block the T current in thalamic neurons30,31,32,33 and also block cloned, expressed human T-type Ca channels, including Ca3.1 to Ca3.3, when applied in clinically relevant concentrations.49 In in vitro studies of spindle rhythms in slices of ferret lateral geniculate nucleus, blocking GABAA-mediated inhibition in these slices is associated with a transition from faster, smaller, spindle-like rhythms to slower, larger, SWD-like rhythms, which are blocked by ethosuximide.142 Huguenard and Prince61 have also demonstrated that in vitro stimulation-evoked thalamic oscillations can be blocked by ethosuximide, a generalized absence anticonvulsant, and that this block is associated with the T-current blocking effects of this drug. Extending these studies to include the cerebral cortex in thin slices of rodent brain maintaining reciprocal connections between thalamus, cortex, and NRT, spontaneous thalamocortical oscillations can be recorded.34 These oscillations are similar in many ways to SWD rhythms and are selectively blocked by drugs effective in the control of generalized absence epilepsy152,153 but not by other anticonvulsants.151 This suggests that thalamocortical circuitry is both necessary and sufficient to generate SWD rhythms, although other areas of the brain are certainly involved in the modulation of these events. Human and animal neuroimaging studies also support the role of both cortex and thalamus in spike wave seizures.2,6,9,10,43,104,123
synaptic circuitry may underlie the generation of normal sleep spindle rhythms and the pathologic thalamocortical rhythms of generalized absence epilepsy. In patients with generalized absence epilepsy, Kellaway71 has demonstrated that the occurrence of SWDs is remarkably correlated with slow-wave sleep stages. In the feline penicillin-generalized epilepsy model of generalized absence epilepsy, SWDs have been shown to evolve from spindle rhythms following intramuscular injection of penicillin. This transition from 7- to 14-Hz spindle rhythms to 3- to 4-Hz SWDs occurs gradually and is accompanied by the behavioral symptoms of absence.47 Consistent with the hypothesis that the T current amplifies and drives both normal and pathologic thalamocortical oscillations is the finding that specific generalized absence anticonvulsants like ethosuximide (Zarontin) and trimethadione (Tridione) block the T current in thalamic neurons30,31,32,33 and also block cloned, expressed human T-type Ca channels, including Ca3.1 to Ca3.3, when applied in clinically relevant concentrations.49 In in vitro studies of spindle rhythms in slices of ferret lateral geniculate nucleus, blocking GABAA-mediated inhibition in these slices is associated with a transition from faster, smaller, spindle-like rhythms to slower, larger, SWD-like rhythms, which are blocked by ethosuximide.142 Huguenard and Prince61 have also demonstrated that in vitro stimulation-evoked thalamic oscillations can be blocked by ethosuximide, a generalized absence anticonvulsant, and that this block is associated with the T-current blocking effects of this drug. Extending these studies to include the cerebral cortex in thin slices of rodent brain maintaining reciprocal connections between thalamus, cortex, and NRT, spontaneous thalamocortical oscillations can be recorded.34 These oscillations are similar in many ways to SWD rhythms and are selectively blocked by drugs effective in the control of generalized absence epilepsy152,153 but not by other anticonvulsants.151 This suggests that thalamocortical circuitry is both necessary and sufficient to generate SWD rhythms, although other areas of the brain are certainly involved in the modulation of these events. Human and animal neuroimaging studies also support the role of both cortex and thalamus in spike wave seizures.2,6,9,10,43,104,123
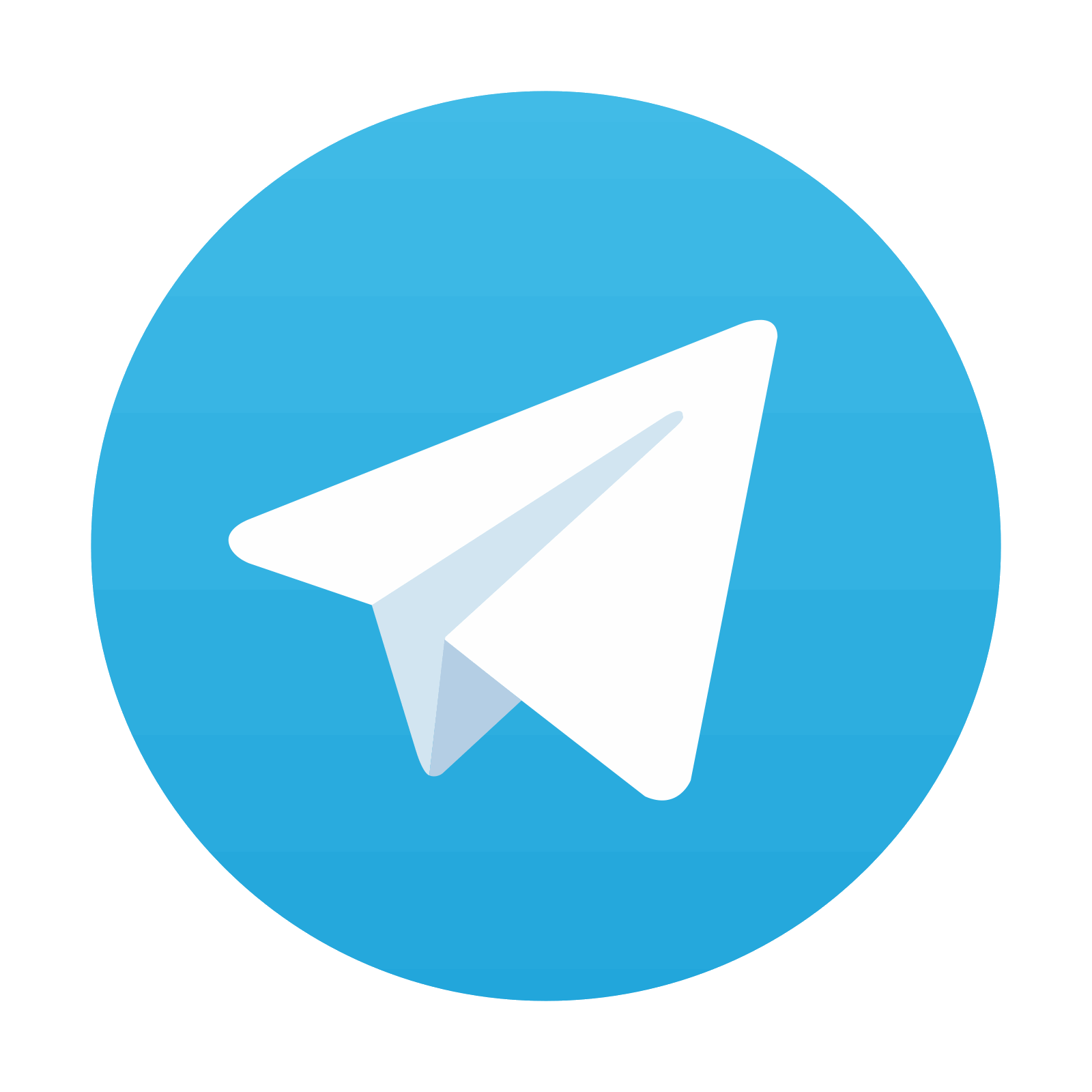
Stay updated, free articles. Join our Telegram channel
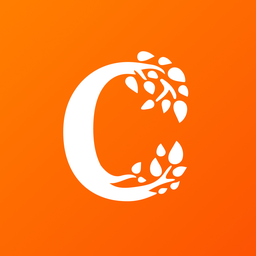
Full access? Get Clinical Tree
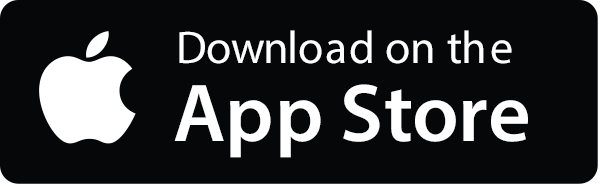
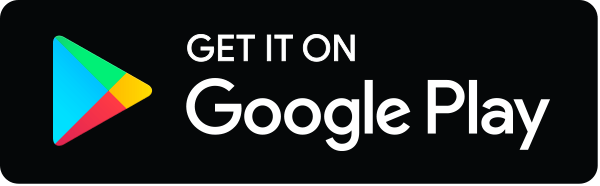