CLINICAL CASE | Friedreich Ataxia
A 10-year-old boy first presented to his pediatrician with difficulty walking. He was an only child, of French-Canadian parents. His parents reported that recently he has difficulty standing still, is constantly shifting his position, and difficulty running. They reported normal motor development initially, but that now he seems clumsy. On examination, he was observed to have a wide-based gait with occasional shifting of position to maintain balance. Sitting and standing were noted to be associated with titubation. He was referred to a child neurologist. After further workup and genetic testing, he was diagnosed with Friedreich ataxia, a progressive spinocerebellar ataxia. Friedreich ataxia is an autosomal recessive disorder, due to a chromosome 9 mutation that, in virtually all cases, is an expansion of a GAA trinucleotide repeat within the gene that codes for the mitochondrial protein frataxin.
He was seen regularly by his neurologist who noted progression in motor signs to include upper extremity ataxia, dysdiadochokinesia, and intention tremor. When asked to maintain a standing posture with his eyes closed, he began to sway and loose his balance (positive Romberg sign). He had no tendon reflexes, such as the knee-jerk or biceps reflexes. Friedreich ataxia patients often have cardiomyopathy, and most patients die as a result of cardiac arrhythmia or congestive heart failure.
Figure 13–1A is an MRI from a young Friedreich ataxia patient. Compared with the MRI from a healthy person (Figure 13–1B), the most notable feature is narrowing of the cervical spinal cord.
Answer the following questions based on your reading of the case and the chapter.
1. Why does the patient have a positive Romberg sign, and how is this related to the loss of tendon reflexes?
2. What could account for the wide-based gait and ataxia?
Key neurological signs and corresponding damaged brain structures Proprioceptive and reflex signsThe cervical spinal cord in the patient is thin compared with a healthy person. Thinning is produced by degeneration of large-diameter somatic sensory afferents; unmyelinated fibers are spared. The degeneration is in the dorsal columns. Whereas the degeneration is accompanied by gliosis, it is insufficient to maintain the normal size of the cord. The dorsal roots show thinning as well. With this large-diameter fiber loss, there is associated loss of tendon reflexes and limb proprioception. Patients with this sensory loss rely on vision to help maintain balance. Often patients have impairment in tactile sensation.
AtaxiaThe loss of limb proprioceptive information leads to incoordination. Further, there is loss of neurons in Clarke’s column, which transmit proprioceptive and other mechanosensory information to the cerebellum; these neurons give rise to the dorsal spinocerebellar tract (Figure 13–6B). Together these factors contribute to ataxia. To compensate for boththe balance impairment and impaired lower extremity coordination, patients adopt a broad-based, slowed gait. Interestingly, Friedreich ataxia patients may not show extensive cerebellar degeneration for most of the course of the disease. Thus, the ataxia may be more related to the somatic sensory loss. In this way, it is similar to motor disturbances in neurosyphilis (see Clinical Case in Chapter 4). Absence of early cerebellar degeneration contrasts with cerebellar degenerative conditions, such as olivopontocerebellar atrophy, which does show extensive degeneration.
FIGURE 13–1
Friedreich ataxia. A. MRI from a person with Friedreich ataxia. Note thinning of the cervical spinal cord (arrow). B. MRI from a healthy person. Note normal cervical spinal cord (lower arrow). Other arrow points to the base of the pons, which contains pontine nuclei that project axons to the cerebellum. (A, Reproduced with permission from Fauci AS, Kasper DL, Braunwald E, et al., eds. Harrison’s Principles of Internal Medicine. 18th ed. New York, NY: McGraw-Hill, Inc; 2012. B, Courtesy of Dr. Joy Hirsch, Columbia University.)

The cerebellum is a fascinating brain structure; more so than many because several of its properties and organizational principles are unexpected. It is a little brain unto itself; roughly half of the neurons in the central nervous system are located in the cerebellum, and when stretched out, it is more than several square meters. That so much of the central nervous system is devoted to the cerebellum means that its functions are particularly important or complex, requiring so much neural power; indeed, it is likely both. It is no surprise that early anatomists termed this structure the cerebellum, which means little brain in Latin. Its microscopic structure is nearly crystalline in its organization, providing insight into a similarity in the way it processes information across this large brain region.
We know that the cerebellum plays a key role in movement, and it does so by regulating the functions of the motor pathways (see Figure 10–2). When this major brain structure is damaged, movements that were smooth and steered accurately become uncoordinated and erratic. Important insights into the general role of the cerebellum in motor control can be gained by considering that the cerebellum receives information from most of the sensory systems and from virtually all other components of the limb and eye movement systems. With these connections, the cerebellum is poised to compare information about the intention of an upcoming movement, by receiving information from the motor pathways, with what actually occurs, by retrieving information from the sensory systems. Research has shown that the cerebellum may compute control signals to correct for differences between intent and action, or errors. The cerebellum, in turn, provides a major input to the brain stem and cortical pathways for limb, trunk, and eye movement control.
The cerebellum also receives information from areas of the central nervous system that do not play a direct role in movement control, such as the parietal association cortex and the limbic association cortex. How is this finding reconciled with its important role in movement control? Many areas of association cortex, as well as higher-order sensory areas, help in the planning of movements—for example, by allowing an individual’s motivation state of being thirsty to influence when to reach for a glass of water or by allowing the determination of the location of the glass to ensure reaching accuracy. However, the cerebellum serves nonmotor functions, too. Indeed, cerebellar damage can produce impairments in language, decision making, and affect that cannot be attributed to motor defects. Contributing to the surprise of the cerebellum, it is involved in many diverse functions, like the central nervous system itself.
Because the three-dimensional organization of the cerebellum is so complex (Figure 13–2), rivaling that of the cerebral hemispheres and diencephalon, its gross anatomy is considered before its functional organization. Located dorsal to the pons and medulla (Figure 13–2A, B), the cerebellum is separated from the overlying cerebral cortex by a tough flap in the dura, the cerebellar tentorium (Figure 13–3B; see Figure 3–15). The inferior surface of the cerebellum is incompletely divided by the posterior cerebellar incisure (Figure 13–2C). The cerebellum comprises an outer cortex containing neuronal cell bodies overlying a region that contains predominantly myelinated axons. The cerebellar cortex contains an extraordinary number of neurons and a rich array of neuron types (see below).
FIGURE 13–2
A. Dorsal view of the brain stem and cerebellum. The borders between the vermis and intermediate and lateral parts of the cerebellar hemisphere are shown. These three parts of the cerebellar cortex also correspond to functional subdivisions. B. The three cerebellar peduncles are revealed when the cerebellum is removed. C. The cerebellum, viewed from the ventral surface. Inset (A) shows lateral view of brain.

FIGURE 13–3
A. Midsagittal cut through the brain, revealing the cerebellar vermis. The inset shows the 10 cerebellar lobules. Lobules I through V comprise the anterior lobe, VI through IX comprise the posterior lobe, and X comprises the flocculonodular lobe. B. Midsagittal MRI, showing the three cerebellar lobes.

Two shallow grooves running from rostral to caudal divide the cerebellar cortex into the vermis, located along the midline, and two hemispheres (Figure 13–2). This anatomical distinction marks the specific functional divisions of the cerebellar cortex (see below). Like the cerebral cortex, the cerebellar cortex is highly convoluted. These characteristic folds, termed folia, are equivalent to the gyri of the cerebral cortex. They vastly increase the amount of cerebellar cortex that can be packed into the posterior cranial fossa (see Figure 3–15).
The cerebellar cortex is organized into groups of folia, termed lobules, that are separated from one another by fissures. In a sagittal section through the vermis, the lobules appear to radiate from the apex of the roof of the fourth ventricle (Figure 13–3A, inset). Anatomists recognize 10 lobules, whose nomenclature is used largely by specialists studying the cerebellum. Two fissures are particularly deep and divide the various lobules into three lobes (Figures 13–2 and 13–3A). The primary fissure separates the anterior lobe from the posterior lobe. The flocculonodular lobe is separated from the posterior lobe by the posterolateral fissure. This lobe consists of the nodulus, located on the midline (equivalent to the vermis of the flocculonodular lobe), and the two flocculi, on either side. The anterior lobe is important in the control of limb and trunk movements, whereas the posterior lobe may be somewhat more important in movement planning and in the nonmotor functions of the cerebellum. The flocculonodular lobe plays a key role in maintaining balance and controlling eye movement.
Beneath the cerebellar cortex is the white matter, which contains axons coursing to and from the cortex (Figure 13–3). The branching pattern of the white matter of the cerebellum inspired early anatomists to refer to it as the arbor vitae (Latin for “tree of life”); hence, the name folia (Latin for “leaves”) rather than gyri, used to describe cerebral cortex convolutions. Embedded within the cerebellar white matter are four bilaterally paired nuclei, the deep cerebellar nuclei: the fastigial nucleus, the globose nucleus, the emboliform nucleus, and the dentate nucleus. The globose and emboliform nuclei are collectively termed the interposed nuclei. These nuclei are shown on the cerebellar cortex surface (Figure 13–2A), as if it were transparent; they are also shown in the schematic transverse section through the pons and cerebellum (Figure 13–4). It is tempting to think that the functional relation between the deep nuclei and cortex of the cerebellum is similar to that of the thalamus and cerebral cortex. But that is wrong; we will see that neurons in the cerebellar cortex send connections to the deep nuclei, not the reverse as with the thalamus and cerebral cortex.
Axons projecting to and from the cerebellum course through the peduncles (Figure 13–2B, C): The superior cerebellar peduncle contains mostly efferent axons, the middle cerebellar peduncle contains only afferent axons, and the inferior cerebellar peduncle contains both afferent and efferent axons. An alternate nomenclature is often used for the cerebellar peduncles in the clinical and scientific literature. The superior cerebellar peduncle is also called the brachium conjunctivum; the middle cerebellar peduncle, the brachium pontis; and the inferior cerebellar peduncle, the restiform body. The various peduncles are distinguished in Figure 13–2B, C because each one has been given a different cut surface in the drawing. Three distinct peduncles would not be apparent had a single cut been drawn.
Figure 13–4 shows the circuits of the cerebellum. There are two major sets of inputs to the cerebellum—termed climbing and mossy fibers—and, with some exceptions, both sets of inputs are directed to neurons in the deep nuclei and cortex. Climbing fibers originate from a single nucleus, the inferior olivary nucleus (see Figure 13–11B); mossy fibers originate from many different brain stem and spinal cord nuclei. In the cortex, climbing fibers synapse on Purkinje neurons, which generate a cerebellar cortex output signal by projecting to neurons in the deep nuclei. Interestingly, many neurons of the vestibular nuclei have similar connections as the deep cerebellar nuclei, receiving inputs from climbing fibers and Purkinje neurons (see below). This points to common developmental origins of the two sets of nuclei.
FIGURE 13–4
Schematic transverse section through the pons and cerebellum, illustrating the salient features of cerebellar circuitry (A). The breakout image to the right shows the basic cerebellar circuit, which is present in all parts of the cerebellar cortex. Open cell bodies and synapses are excitatory and filled cell bodies and synapses are inhibitory. Part B shows the location of the deep cerebellar nuclei. The vestibular nuclei are also shown because they are anatomically equivalent to the deep cerebellar nuclei for the flocculonodular lobe. They receive inputs from Purkinje cells and the inferior olivary nucleus.

Mossy fibers engage a network of cerebellar excitatory and inhibitory interneurons (Figure 13–4A). The excitatory interneurons, in turn, synapse on Purkinje neurons, also leading to a cerebellar cortex output signal. The inhibitory interneurons help to regulate Purkinje neurons activity, making it easier—with less inhibition—or harder with more, for the climbing and mossy fibers to generate a cortical output signal. Surprisingly, the Purkinje neuron is an inhibitory projection neuron.
The cerebellum has three functional divisions, each consisting of a portion of the cerebellar cortex and one or more deep nuclei (Figures 13–2 and 13–5). Each functional division of the cerebellum uses the same basic circuit to carry out its own tasks, the circuit shown in Figure 13–4A. However, each functional division differs from the others with respect to the specific input sources and the specific structures to which it projects. The divisions are named for their major sources of information:
The spinocerebellum, which receives highly organized somatic sensory inputs from the spinal cord, is important in controlling the posture and movements of the trunk and limbs (Figure 13–5A). The spinocerebellum comprises the vermis; the adjoining intermediate hemisphere, of both the anterior and posterior lobes; and the fastigial and interposed nuclei. This division also receives information from structures other than the spinal cord; a portion receives trigeminal and other sensory cranial nerve information. A portion of the vermis of the posterior lobe may play a role in nonmotor functions.
The cerebrocerebellum, which receives input indirectly from the cerebral cortex, participates in the planning of movement and nonmotor functions. This division consists of the lateral hemisphere, in both the anterior and posterior lobes, and the dentate nucleus.
The vestibulocerebellum, which receives input directly from the vestibular labyrinth as well as the vestibular nuclei, helps in maintaining balance and controlling head and eye movements. This cerebellar division corresponds to the flocculonodular lobe. There is no deep cerebellar nucleus for the vestibulocerebellum. Instead, the vestibular nuclei serve a similar role.
FIGURE 13–5
The anatomy (A) and three functional divisions (B) are shown in schematic views of the cerebellum. The topographic organization of somatic sensory inputs to the spinocerebellum is also shown in A. These inputs are somatotopically organized. Visual, auditory, and vestibular inputs are directed predominantly to the “head” areas. The deep cerebellar nuclei are shaded dark brown. The nuclei are labeled in Figure 13-2. Inset shows how the schematic “flattened out” view is constructed. (Inset in A modified from Kandel E, Schwartz JH, Jessell T, eds. Principles of Neural Science. 4th ed. New York, NY: McGraw-Hill.)

The spinocerebellum is important in the control of body musculature. It is somatotopically organized: The vermis controls axial and proximal muscles and the intermediate hemisphere, limb muscles (Figure 13–5A). This mediolateral somatotopic arrangement recalls the somatotopic organization of the ventral horn, where medial motor neurons innervate axial and proximal limb muscles, and those located laterally innervate more distal muscles (see Figure 10–3). As with the descending motor pathways, the components of the spinocerebellum that control the distal limb are predominantly crossed, whereas the components controlling proximal and axial muscles have a more bilateral organization.
The organization of the spinocerebellum that captures both its salient and clinical features (see later section on the motor effects of cerebellar damage) is shown in Figure 13–6. It receives somatic sensory information primarily from mechanoreceptors, especially those that innervate muscle (see Table 4–1), from the limbs and trunk, via ipsilateral spinocerebellar tracts. The dorsal spinocerebellar tract originates from Clarke’s nucleus and transmits sensory information from the leg and lower trunk to the cerebellar nuclei and cortex. By contrast, the cuneocerebellar tract originates from the accessory cuneate nucleus. It transmits sensory information from the arm and upper trunk. Several cerebellar conditions, including Fredrich ataxia, produce limb and trunk control impairments by causing degeneration in these ascending cerebellar pathways (see clinical case in this chapter). Ataxia is a kind of incoordination that occurs following many different kinds of cerebellar disease.
FIGURE 13–6
Key features of the input-output organization of the lateral spinocerebellum, which is important for controlling the limbs. Part A shows the dorsal and cuneocerebellar tracts projecting to the cerebellum (lower four sections). The upper sections show the cerebellar output path. Part B shows the ventral and rostral spinocerebellar tracts. The output of the lateral spinocerebellum is shown in A. The inset shows the cerebellar cortex and deep nuclei; the lateral spinocerebellum is highlighted.

The axons of both of these tracts travel through the inferior cerebellar peduncle (Figure 13–6); they are examples of mossy fibers, forming connections with diverse cerebellar neurons. For limb control, the spinocerebellar axons synapse on neurons in the interposed nuclei. Information also projects to neurons of the intermediate hemisphere of the cerebellar cortex, where Purkinje neurons project to the interposed nuclei (Figure 13–5B). The interposed nuclei project from the cerebellum, through the superior cerebellar peduncle, to the magnocellular component of the red nucleus and, predominantly, via the ventrolateral nucleus of the thalamus, to motor areas of the frontal lobe (Figures 13–3B). These components of the motor systems give rise to the lateral descending pathways: the rubrospinal and lateral corticospinal tracts. The logic of the laterality of these connections ensures that somatic sensory information from one limb is projected to the side of the spinocerebellum that, in turns, projects to the parts of the lateral motor pathways that control the same limb.
For proximal muscle control, including the trunk and upper back, the circuitry is more bilaterally organized (Figures 13–5B and 13–7). Here the dorsal spinocerebellar and cuneocerebellar tracts project both to the fastigial nuclei and the vermis of the cerebellar cortex, which, in turn, also projects to the fastigial nuclei (Figure 13–7). This deep nucleus influences motor neurons primarily through their projections onto the medial descending pathways, the reticulospinal (Figure 13–7) and vestibulospinal tracts (Figure 12–3B). The fastigial nucleus has also a small ascending projection, via a thalamic relay, to cells of origin of the ventral corticospinal tract in primary and premotor cortical areas. This efferent projection exits through the superior cerebellar peduncle. Some Purkinje neurons of the vermis send their axons to the vestibular nuclei (see following section on vestibulocerebellum).
Two other spinocerebellar pathways (Figure 13–6B,)—the ventral and rostral spinocerebellar tracts, for the lower and upper halves of the body—are thought to transmit internal feedback signals for correcting inaccurate movements rather than somatic sensory information. Many of the axons that decussate in the spinal cord cross again in the superior cerebellar peduncle to terminate in the ipsilateral cerebellum; these axons are “doubly crossed.” There are also trigeminocerebellar pathways that originate from the spinal trigeminal nucleus, principally from parts of the interpolar and oral nuclei (see Chapter 6).
The cerebrocerebellum (Figures 13–5B and 13–8) is primarily involved in the planning of movement and is interconnected with diverse regions of the cerebral cortex. The major input to the cerebrocerebellum is from the contralateral cerebral cortex, not only from the motor areas but also from the sensory and association areas (Figure 13–8). This projection is relayed by neurons in the ipsilateral pontine nuclei (Figure 13–8A, and inset). The pontine nuclei, in turn, project to the contralateral cerebellar cortex through the middle cerebellar peduncle. Purkinje neurons of the cerebrocerebellum project to the dentate nucleus, the largest and most lateral of the deep nuclei. Neurons in the dentate nucleus project their axons to two main motor control centers. The first is the motor relay nucleus of the thalamus, the ventrolateral nucleus, and from here, to the primary motor cortex and premotor areas. The logic of the laterality of these connections is that the cerebral cortex controlling one limb also projects to the cerebrocerebellum controlling the same limb.
FIGURE 13–8
Afferent and efferent connections of the cerebrocerebellum (A) and the portion of the cerebellar cortex that corresponds to this cerebellar division (B). Note that the major input to the pontine nuclei is from large areas of the cerebral cortex, although input from only a single site is shown. Most cortical regions project to the cerebellum, via the pontine nuclei (see inset). Different cortical areas project to distinct sets of pontine nuclei, represented as different shades in the schematic inset of ventral pons. The darkest gray areas correspond to descending cortical axons. (Adapted from Schmahmann JD, Pandya DN. The cerebrocerebellar system. Int Rev Neurobiol. 1997;41:31-60.)
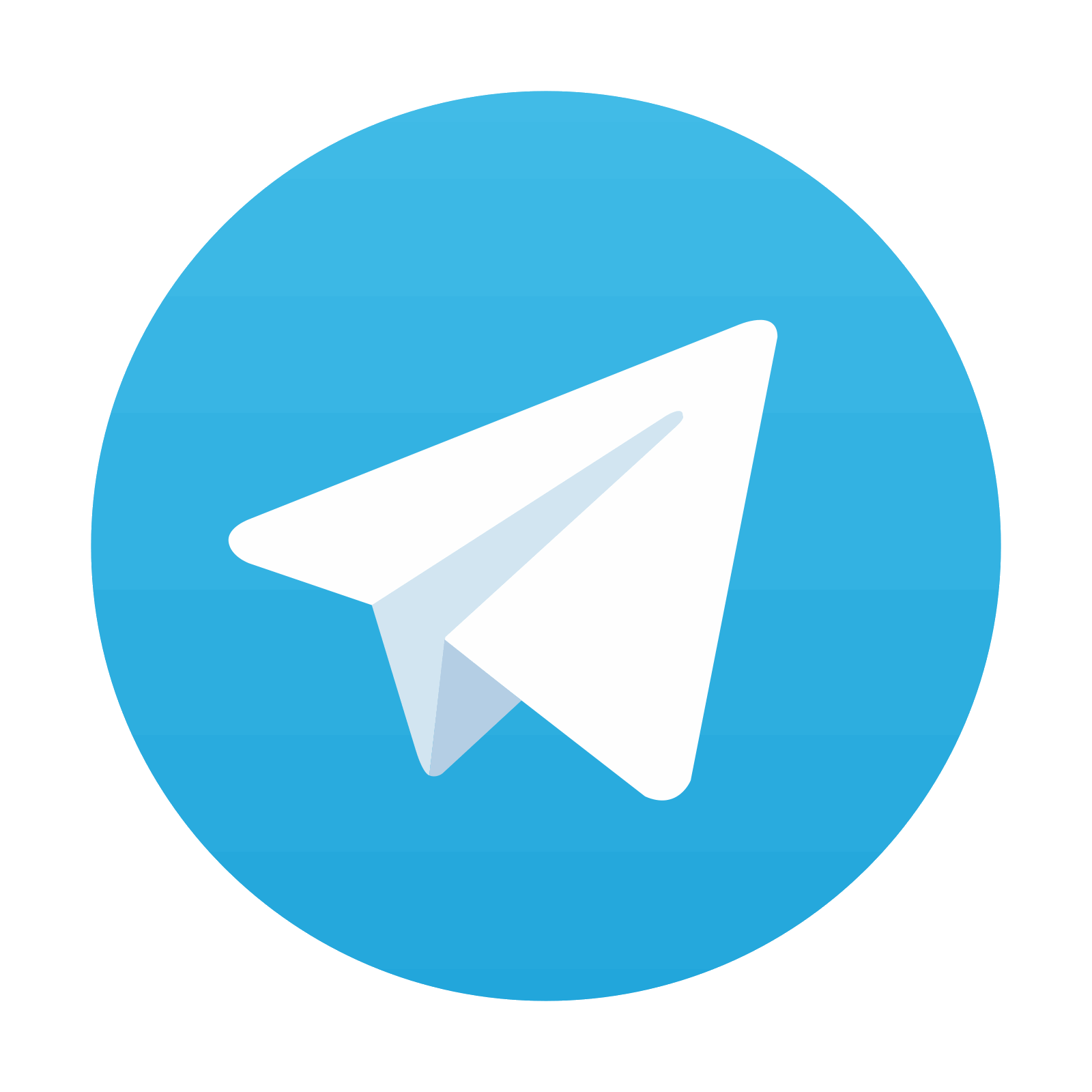
Stay updated, free articles. Join our Telegram channel
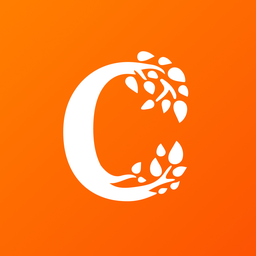
Full access? Get Clinical Tree
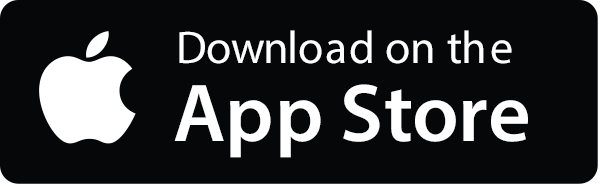
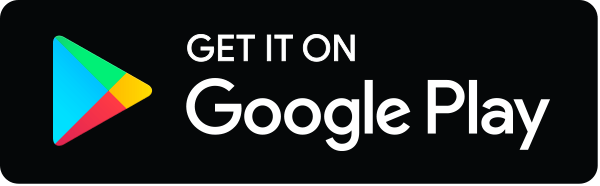
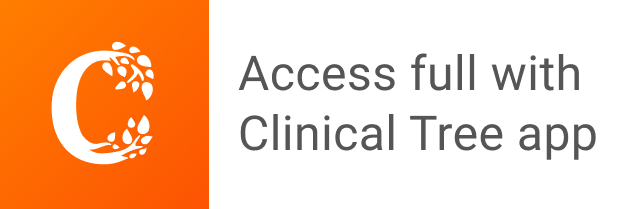