Fig. 11.1
Toxic and protective events affected by MSCs and their time course after TBI
11.2.1 Trophic Factors
There is experimental evidence of beneficial effects in tissue repair and regeneration after transplantation of MSCs despite a rare/transient presence of transplanted cells in the host tissues. This prompted the proposal that the restorative outcome might be due to mechanisms other than in vivo differentiation of injected cells and replacement of host defective cells (Gnecchi et al. 2005, 2008; Caplan and Dennis 2006; Meirelles and Nardi 2009; de Girolamo et al. 2013; Neirinckx et al. 2013b). Thus a new mechanism was proposed in which the transplanted MSCs release bioactive trophic molecules that might reprogram the surrounding host environment through paracrine actions. In support of this, it has been shown that the conditioned medium generated from the culture of MSCs (i.e. the medium containing the set of molecules secreted by these cells) injected in animal models of disease can recapitulate the beneficial effects of their cellular counterpart in tissue protection and repair (Timmers et al. 2007; van Poll et al. 2008; Di Santo et al. 2009; Cargnoni et al. 2012, 2014).
Several MSC secreted pleiotropic molecules are involved in these effects, including cytokines/chemokines, growth factors, extracellular matrix proteins and tissue remodelling enzymes (Caplan 2009). In vitro studies have described the secretome profile of MSCs isolated from BM, the best characterized source (Kinnaird et al. 2004; Schinköthe et al. 2008; Tate et al. 2010). Among the secreted factors released in vitro under basal conditions, neurotrophins i.e. brain-derived neurotrophic factor (BDNF), glial cell-derived neurotrophic factor (GDNF), nerve growth factor (NGF) and neurotrophin−3 (NT−3) are of great interest for TBI (Arnhold et al. 2006; Crigler et al. 2006). In vivo studies have investigated the effect of MSCs on neurotrophin production after TBI, confirming that these neurotrophins increase after MSC treatment in the injured brain. The question remains, however, whether the infused cells are directly or indirectly responsible for the changes. Commonly used biochemical methods (western blot or ELISA) cannot distinguish between the human (in case of xenotransplant) and the rodent isoforms of neurotrophins, so when measuring these proteins after TBI and MSC treatment, it is not possible to distinguish the contribution of infused MSCs from that of host cells. Thus, increased production could be related to direct secretion by MSCs and/or to an indirect effect of MSCs on endogenous cell populations.
Kim et al. (2010) injected 2 million-human MSCs intravenously 24 h after TBI, modeled by controlled cortical impact (CCI) in rats. At 48 h the homogenized injured hemisphere of TBI MSC rats showed increased amounts of NGF, BDNF and NT−3. NGF plays a key role in neuronal plasticity and prevents neuronal apoptosis (Saito et al. 2004). BDNF is involved in neural development (Ernfors et al. 1995), neurogenesis (Zigova et al. 1998; Benraiss et al. 2001) and in synaptic plasticity processes (Parkhurst et al. 2013). NT−3 supports the survival and differentiation of existing neurons and induces neurite outgrowth (Kumar et al. 1998; Bregman et al. 2002). The survival cellular signaling of these neurotrophins is mediated by binding to the neurotrophic tyrosine kinase receptor (Trk). NGF preferentially binds TrkA, BNDF binds TrkB and NT−3 binds TrkC (Chao 2003). Autophosphorylation of the cytosolic domain induces the phosphorylation of Akt and ERK signal transducers which, in turn, through NF-κB, induces the transcription of genes responsible for cell survival (Yuan and Yankner 2000; Chao 2003). The increased expression of neurotrophins in TBI MSC treated animals found by Kim et al. was in fact related to increased phosphorylation of Akt (but not of ERK) at 2 days, together with reduced cleavage of caspase−3 (the main signal for cell apoptosis) at 8 days. The molecular modifications induced by MSCs resulted in the functional recovery of motor function (Kim et al. 2010).
Our group and others have reported similar results on the increase in neurotrophin production with intravenous (iv) (Mahmood et al. 2004a) or intracerebroventricular (icv) (Chen et al. 2005; Zanier et al. 2011) transplantation of MSCs after TBI, showing increased production of BDNF (Mahmood et al. 2004a; Zanier et al. 2011) or NGF (Mahmood et al. 2004a; Chen et al. 2005) induced by infused/endogenous cells. We used MSCs from human cord blood, observing for the first time an effect on neurotrophin production similar to that described with BM-MSCs (Zanier et al. 2011).
In addition to the direct and indirect production of neurotrophins, a third mechanism for the increase in neurotrophins induced by MSCs was shown by Mahmood et al. (Mahmood et al. 2011). Three millions human MSCs transplanted 7 days after CCI, directly in the lesion cavity or impregnated in scaffolds, increased the production of tissue plasminogen activator (tPA) in the boundary zone 15 days after surgery. tPA regulates the final production of plasmin, a serine protease known for its part in the degradation of fibrin blood clots. In the brain, plasmin plays a pivotal role in the activation of BDNF and NGF, by cleaving pro-BDNF and pro-NGF into active forms (Bruno and Cuello 2006; Barnes and Thomas 2008). Thus, focusing on the increase in neurotrophins, MSCs may: (1) directly produce and/or (2) induce other cells to produce and/or (3) produce, or induce the production of, proteases that can cleave neurotrophin precursors. In principle the paracrine action means that trophic factors alone can be applied, although up to now single-drug therapy has always failed in clinical trials . MSCs act like poly-pharmacy and improve the neurological outcome by creating a growth-promoting niche, this pleiotropic activity more than any single released factor possibly being the key to MSC therapeutic efficacy.
11.2.2 Microvesicles
MSCs may also exert their paracrine effects through the release of extracellular membrane vesicles that mediate the horizontal transfer of signals and molecules from one cell to another even over long distances. MSCs, like other many cell types (Camussi et al. 2010), release circular membrane fragments known as microvesicles, either constitutively or in response to activation stimuli. These microvesicles, depending on their size and process of release, are generally classified as exosomes (multivesicular bodies of 30–120 nm and released from the endosomal compartment) or shedding vesicles (100–1000 nm, derived from direct budding of the cell plasma membrane). They are capable of transferring specific proteins, lipids and nucleic acids (mRNA, microRNA and DNA) thus serving as a unique mechanism for the intercellular trafficking of complex biological messages (Verderio 2013). However, the complete biochemical composition of microvesicles remains to be determined and seems to vary depending on the cell source (Camussi et al. 2010). Recent studies have reported on the miRNA (Collino et al. 2010) and protein (Kim et al. 2012) composition of microvesicles from human BM-MSCs, indicating that they include several molecules representative of the multiple properties of MSCs.
In spite of the fact that the physiological role and the biogenesis of MSC-derived microvesicles are still only partially understood, they appear to have a vital role in cell-to-cell communication (Mathivanan et al. 2010). In terms of the microvesicle-mediated therapeutic effects of MSCs, human MSC-derived microvesicles injected in SCID mice with glycerol-induced acute kidney injury (AKI) had the same efficacy as their cellular counterparts on the functional and morphological recovery of AKI (Bruno et al. 2009) and protected SCID mice from lethal cisplatin-induced AKI (Bruno et al. 2012). Exosomes released by human umbilical cord blood MSCs were reported to alleviate carbon tetrachloride (CCl4)-induced fibrotic liver (Li et al. 2013). Lai et al. have documented the beneficial effects after the injection of MSC-derived microvesicles in animal models with cardiovascular diseases (Lai et al. 2010, 2011, 2013; Arslan et al. 2013). Zhu and collaborators, have reported that human BM-MSC-derived microvesicles were therapeutically effective in C57BL/6 mice with E.coli endotoxin-induced acute lung injury (Zhu et al. 2014). Microvesicles have also been used to deliver selected factors and target specific therapeutic signals. For instance, Katsuda and collaborators administered adipose tissue-derived MSC exosomes carrying enzymatically active neprilysin, a trivial beta amyloid-degrading enzyme in Alzheimer rodents (Katsuda et al. 2013). Exosomes were transferred into N2a cells overproducing Aβ and seemed to reduce both secreted and intracellular Aβ levels, suggesting therapeutic possibilities for adipose tissue-derived MSC exosomes in Alzheimer disease.
Alvarez-Erviti and collaborators succeeded in delivering functional siRNA to the mouse brain by systemically injecting targeted exosomes (Alvarez-Erviti et al. 2011). Xin et al. have reported that systemic administration of rat BM-MSC-derived exosomes after acute brain injury in ischemic rats (induced by middle cerebral artery occlusion) significantly improved functional recovery compared with control rats (Xin et al. 2013a) and raised miR−133b in the ipsilateral hemisphere.
It was shown earlier that miR−133b is specifically expressed in midbrain dopaminergic neurons and regulates the production of tyrosine hydroxylase and the dopamine transporter (Dreyer 2010). Employing knock-in and knock-down technologies to up- or down-regulate miR−133b, evidence was obtained that exosomes from MSCs mediate the miR−133b transfer to astrocytes and neurons, which in turn regulates gene expression and is involved in neurite remodeling and functional recovery after stroke (Xin et al. 2013b). The bi-lipid membrane composition enriched with membrane-bound proteins suggests that microvesicles may become a therapeutic agent, homing the injured brain and treating TBI. To our knowledge the effects of microvesicles generated by MSCs after TBI have not been examined and detailed studies are needed to see whether MSC-derived microvesicles mimic the phenotype of their parent cells and provide a protective effect on TBI.
11.2.3 Immunomodulation
After TBI, the inflammatory response plays a major role in the evolution of the brain lesion. The innate immune response is needed for resolution of the damage and wound healing but it is also a key factor in the secondary injury cascades leading to exacerbation of the initial damage . The blood brain barrier (BBB) breakdown allows the passage of inflammatory molecules and cells into the injured parenchyma. The infiltrated leukocytes directly affect neuronal survival, releasing pro-inflammatory cytokines, reactive oxygen species (ROS), nitric oxide (NO) and cytotoxic proteases which may in turn exacerbate neuronal death (Lucas et al. 2006; Morganti-Kossmann et al. 2007). However, inflammatory mediators and immune cells can also have a neuroprotective effect and promote neurogenesis and lesion repair after central nervous system (CNS) injuries (Correale and Villa 2004). Recruited macrophages and activated microglia have been proposed as beneficial through different mechanisms including glutamate uptake (Nakajima et al. 2008), removal of cell debris (Stoll and Jander 1999) and production of neurotrophins (Batchelor et al. 1999; David and Kroner 2011). Thus strategies to direct the inflammatory response toward a protective phenotype could prove effective for TBI patients.
MSCs exert immunomodulatory properties : several studies have demonstrated that they influence the function of different immune cells in vitro, including proliferation of T cells after stimulation by alloantigens or mitogens, as well as activation of T cells by CD3 and CD28 antibodies. MSCs inhibit the generation and function of monocyte-derived dendritic cells in vitro and may also modulate B-cell functions, affecting the cytotoxic activity of natural killer cells by inhibiting proliferation and cytokine secretion (reviewed in Uccelli et al. 2008) .
Despite the general agreement on the MSC’s effects on the immune cell functions, little is known about the mechanisms involved. For example, inhibition of T-cell proliferation by MSCs appears to depend on cell-to-cell contact and on the release of soluble factors. MSC-derived molecules that may have immunomodulatory activity on T-cell responses include transforming growth factor-β (TGF-β), indoleamine 2,3-dioxygenase (IDO), prostaglandin E2 (PGE−2), interleukin (IL)-10, NO, miRNA (reviewed in Uccelli et al. 2008), as well as exosomes (see above). However, this aspect of the MSC biology calls for further study. Characterization of the composition and functions of the MSC secretome will undoubtedly provide important information towards a better understanding.
In TBI pathology, the effects of MSCs on immune responses have been investigated by different groups. Zhang et al. (2013) transplanted 4 million syngenic MSCs intravenously 2 h after weight-drop injury in rats, reducing the infiltration of neutrophils (MPO+) and CD3+ lymphocytes and the infiltration/activation of macrophages/microglia (Iba+) 3 days after injury . These anti-inflammatory effects were accompanied by a reduction of apoptotic cells in the pericontusional cortex and early improvement of sensorimotor function. Analysis of cytokine levels in the injured cortex showed decreases in the expression of the pro-inflammatory mediators IL−1β, IL−6, IL−17, tumor necrosis factor-α (TNF-α) and interferon (INF)-γ in the acute phase (12–72h) and increased expression of the anti-inflammatory cytokines IL−10 and TGF-β1 24–72h after TBI, indicating attenuation of the inflammatory response and a shift toward an anti-inflammatory microenvironment. There was also lower expression of the chemoattractant mediators monocyte chemotactic protein−1 (MCP−1, also known as CCL2, chemoattractant for monocytes, macrophages and microglia), macrophage inflammatory protein−2 (MIP−2, also known as CXCL2, secreted by monocytes/macrophages is chemotactic for polymorphonuclear leukocytes (Wolpe et al. 1989)) and RANTES (also known as CCL5, chemotactic for T cells, eosinophils and basophils and active in recruiting leukocytes into inflammatory sites), all indicating less recruitment of inflammatory cells from the circulatory system. The authors indicated the tumor necrosis factor-stimulated gene−6 (TSG−6) as one possible mediator of the attenuated innate immune response after MSC treatment. TSG−6 is an anti-inflammatory protein induced by TNFα and IL−1 (Wisniewski and Vilcek 1997) and was up-regulated in MSC-treated rats, together with a decrease in the expression of NF-kB, an important transcription factor that regulates many genes involved in the inflammatory response. Similarly, Watanabe et al. focused on TSG−6 and MSC-mediated protection (Watanabe et al. 2013). CCI injured mice were injected with 10 million MSCs or TSG−6 protein (50 µg/mouse) intravenously 6 h after TBI. Both treatments induced a comparable inhibition of neutrophil infiltration and a decrease in the expression of matrix metalloproteinase (MMP)-9 in the injured cortex at 24 h, leading to less BBB leakage on day three. These data support the idea that MSC are able to attenuate the inflammatory response and one possible mediator is TSG−6 .
Another important MSC-mediated immunomodulatory effect is the ability to modulate the activation state of microglia/macrophages (M, resident or infiltrated immune cells in the brain) toward a beneficial and protective phenotype after cerebral insults. There is growing evidence that M play ambivalent roles in inflammation (Lai and Todd 2008; Madinier et al. 2009; Shechter and Schwartz 2013). In response to TBI, M can adopt diverse, complex activation states, enabling them to participate in the cytotoxic response, but also in immune regulation and injury resolution (Kigerl et al. 2009; Longhi et al. 2013; Shechter and Schwartz 2013).
The activation states of M can be classified in two main phenotypes: classical activation, or M1, with pro-inflammatory and detrimental properties, and the alternative one, M2, with beneficial and protective functions . Among M2-activated M, three subsets can be distinguished with different properties: M2a with pro-regenerative functions (mainly growth stimulation and tissue repair), M2b with immunoregulatory phenotype, and M2c involved in debris scavenging and healing functions (David and Kroner 2011). After acute brain injury, both M1 and M2 activation states are present in the injured tissue (Perego et al. 2011; Hu et al. 2012); however, the M2 phenotype vanishes very soon, favoring the balance towards the M1 phenotype which is responsible for the continued production of pro-inflammatory cytokines and exacerbation of injury (Loane and Byrnes 2010). Great attention has been centered on MSC’s effects on M activation in different in vitro or in vivo models (Maggini et al. 2010; Ooi et al. 2010; Nakajima et al. 2012; Song et al. 2013), with the aim to skew pro-inflammatory M1 polarization towards M with an M2 immunosuppressive and pro-regenerative profile. After TBI, Walker et al. (2012a) reported that iv injection of human BM-derived multipotent adult progenitor cells (MAPC, 107/kg, two injections 2 and 24 h after CCI) raised the percentage of T regulatory cells in the periphery (spleen and blood) and the ratio of M2/M1 microglia in the brain. They reported that direct contact between MAPC and splenocytes is required for modulation of parenchymal microglia and attributed a central role to extraneurologic organs, by which transplanted cells act as “remote bioreactors” that boost systemic anti-inflammatory cytokine production, thereby affecting the resident microglia and the infiltrated macrophages in the peri-injury area (Walker et al. 2012b). Our group has demonstrated that MSCs can also act as a “local bioreactor” in the brain (Zanier et al. 2014). MSCs injected icv 24 h after CCI induced early and lasting acquisition of protective M2 traits by M. In the absence of any treatment the M2 wave seen 3 days after TBI vanished very soon, returning to basal levels 7 days after injury, while after MSC treatment the significant increase of M2 traits at 3 days was still present 7 days after injury. MSC-treated animals also showed a decrease in active phagocytosis of the M populations in the perilesional cortex 7 days after injury .
The role of phagocytosis after an acute brain injury is complex. On one hand, phagocytosis is needed to remove cell debris and dying cells, thus limiting the propagation of danger signals that can exacerbate damage progression (“secondary phagocytosis”). On the other hand, under certain conditions such as inflammation , phagocytosis can target viable neurons, causing their death (“primary phagocytosis”). After injury, such detrimental phagocytic activity may result from exposure of eat-me signals on otherwise viable neurons as a result of subtoxic and reversible insult (Neher et al. 2011, 2012). The ability of MSCs to influence and limit “primary phagocytosis” might be a key pathway to confer protection after TBI.
A direct link between MSC infusion and changes in the activation state of microglia was established in vitro by us and others (Giunti et al. 2012). Primary microglia cultures were exposed to an inflammatory condition, followed 2 h later by the addition of MSCs. Pro-inflammatory stimuli induced microglia M1 polarization, while the co-culture condition was able to skew the M1 acquired phenotype towards M2 polarization.
These data indicate that immunomodulation is a real healing/protective mechanism of action of MSCs after TBI, involving modulation of both the local and systemic inflammatory responses .
11.2.4 Brain Repair
The aim of repair is to rewire or restore the damaged or missing parenchyma, yielding new functional tissue. Brain repair can be considered as the ability of the CNS to remodel itself in response to insults that impair tissue homeostasis. Beside damage, TBI induces, endogenous neurorestorative events (Richardson et al. 2007). The brain retains neurogenic zones with neural stem cells that can differentiate into functional neurons (Kokaia and Lindvall 2003; Zhao et al. 2008). Like neurogenesis, other endogenous restorative processes take place in the traumatized brain and include gliogenesis, angiogenesis, synaptic plasticity and axonal sprouting. All these events: (1) are stimulated by endogenous growth factors, (2) may continue for weeks and months, (3) may contribute to functional and structural recovery. However, these spontaneous brain restorative processes are largely ineffective in counteracting the progression of damage and cumulative increases in injury over time. Providing the injured tissue with a facilitatory milieu that enhances neuroregeneration is an important additional therapeutic strategy. The roles of MSCs in neurogenesis, synaptic plasticity and axonal sprouting and in angiogenesis/vascular repair are discussed under the heading below.
11.2.4.1 Neurogenesis
The beneficial effects on functional and structural damage after MSC infusion in TBI models are not the result of replacement of injured or dead cells with exogenous cells. The first experimental study using MSCs for TBI was published in 2001 by Chopp and collaborators (Lu et al. 2001b) and showed that MSCs infused intravenously 24 h after TBI improved motor function in rats. Analysis of their fate showed that less than 0.005 % of transplanted MSCs migrated to the injured cerebral area and only 5 % expressed the neuronal marker NeuN and 7 % the astrocytic marker glial fibrillary acidic protein (GFAP). The same group then worked to increase the engraftment rate in the traumatically injured area by intra-arterial (ia) (Lu et al. 2001a) or intracerebral (ic) (Mahmood et al. 2002) injection or by culturing MSCs with neurotrophic factors (Lu et al. 2001a). In vivo neuronal differentiation occurred in a negligible number of MSCs, with no relationship between route of administration and degree of efficacy. In the context of TBI no evidence has ever been provided that neurons newly generated from MSCs become functionally active. In some studies functional improvement was induced by MSCs in the absence of any neuronal differentiation (Mahmood et al. 2005; Mori et al. 2005) and there is ample evidence that MSC-treated animals enjoy long lasting protection even when no transplanted cells are found in the brain injured tissue (Chen et al. 2005; Kim et al. 2010; Zanier et al. 2011; Pischiutta et al. 2014). These findings, along with evidence that transplanted MSCs induce early (days-weeks) functional improvement suggest that the beneficial effects are due to stimulation of endogenous neuroreparative processes through a paracrine action, so neuron replacement is not the primary mechanism of MSC-induced protection.
The observation that MSCs may promote endogenous restorative processes through interaction with local neural cells is consistent with several studies showing the induction of local neurogenesis after MSC infusion in acute brain injured rodents. After TBI we found greater expression of doublecortin (DCX, a marker of developing neurons) in the subventricular zone (svz) of MSC-treated than in untreated TBI mice at 5 weeks post-injury (Pischiutta et al. 2014). Similarly, Mahmood et al. found an increased proliferation rate (5-bromo−2-deoxyuridine (BrdU) positive cells) in the svz and subgranular zone (sgz) of traumatized rats treated with MSCs (Mahmood et al. 2004b). This effect was regularly detected after iv, ic or icv MSCs (Mahmood et al. 2004b).
11.2.4.2 Synaptic Plasticity and Axonal Sprouting
Neuroplasticity is a major compensatory mechanism following acute brain injury. Through axonal sprouting, undamaged axons can reconnect neurons whose links were injured or disrupted or can establish new networks with undamaged neurons. Thus adjacent viable regions of the cortex might function vicariously after injury (Nudo 2013). The MSC’s ability to foster synaptic processes after TBI was demonstrated by infusing a fluorescent dye (DiI) into the contralateral cortex 5 weeks after injury and measuring its transport from the injection site to the injured hemisphere through the corpus callosum 1 week later (Xiong et al. 2009). MSC-treated animals had a greater axonal fiber length that was directly correlated to performance in behavioral tests, indicating that neuroplastic processes are enhanced by MSCs, and can promote neuronal connectivity by directing axonal projections, neurite outgrowth and elongation in the injured cortex. Reduction of the growth inhibitory molecule Nogo-A is linked to MSC promotion of neuroplastic processes (Mahmood et al. 2013a). Nogo-A is a myelin-derived inhibitor of axonal outgrowth highly expressed in scar tissue after TBI (Yang et al. 2013). Acute glial activation is needed to clear excessive glutamate release and remove dying cells and cellular debris after injury, limiting damage progression (Laird et al. 2008; Loane and Byrnes 2010). However, excessive glial scar in chronic stages inhibits remodeling (Iseki et al. 2012; Lin et al. 2014). Our group has demonstrated that both cord blood (Zanier et al. 2011) and BM (Pischiutta et al. 2014) derived MSCs inhibit glial scar formation around the traumatized area 5 weeks after injury, with smaller lesion volume and better functional recovery. Thus, inhibition of gliotic scar and reduction of inhibitory molecules by MSCs render the damaged tissue more permissive to neuroregenerative and neuroplastic processes, fostering recovery and better outcome. Whether MSCs are directly involved in promoting plasticity of the injured neurons and proliferation of the endogenous cells in the subventricular zone or whether this is due to the interaction between MSCs and glial cells which, in turn, will be induced to secrete neurotrophins, still needs to be clarified.
11.2.4.3 Angiogenesis/Vascular Repair
The human brain accounts for 2 % of body weight but receives about 20 % of cardiac output and uses about 20 % of oxygen. Brain glycogen reserves are very limited so cerebral metabolism depends strictly on blood flow and energy supply. Deficient delivery of oxygen, glucose and nutrients increases susceptibility to neuronal injury. Global and regional cerebral ischemia/hypoperfusion have been observed in animal models of TBI and in brain-injured patients, after analysis of post-mortem tissue (Jones et al. 1981; Graham et al. 1989; Jenkins et al. 1989). Therapeutic strategies to restore blood flow and brain oxygenation are therefore particularly important for the vulnerable perilesional tissue that can be preserved from secondary damage and death if oxygen and glucose delivery is adequate.
Strategies aimed at restoring cerebral blood flow (CBF) after TBI may act on different mechanisms including vascular preservation from secondary damage and angiogenesis/vascular remodeling. MSC effects on CBF have been examined in live animals by magnetic resonance imaging (MRI) (Li et al. 2011). CBF was quantified by arterial spin-labeling done longitudinally on rats up to 6 weeks post-TBI. TBI induced an early, persistent reduction of CBF (< 30 mL/100 g/min) in the mechanically damaged and remote regions. MSCs restored and preserved CBF in the brain regions adjacent to and further from the lesion at chronic stages (3–6 weeks), showing their ability to improve hemodynamics and moderate post-TBI hypoperfusion. Post-mortem analysis showed MSCs boosted vascular density in the pericontusional area when administered in the acute phase (24 h) (Pischiutta et al. 2014; Mahmood et al. 2007; Qu et al. 2008), or in the sub-acute phase (7 days) (Qu et al. 2009) after TBI and even at 2 months (Bonilla et al. 2009). In principle a rescue effect on pre-existing vessels and/or the promotion of neoangiogenic processes may be responsible for the increase in vessel density, though the effects after delayed administration of MSCs clearly support a regenerative action on brain vasculature. Gene expression microarray analysis on MSCs in vitro detected the expression of genes involved in angiogenic processes that could potentially sustain both neurovascular repair at early stages and neovascularization later (Qu et al. 2011). The vascular network improved when MSCs were transplanted ic (Qu et al. 2008; Bonilla et al. 2009) or icv (Pischiutta et al. 2014) suggesting a paracrine effects of infused MSCs. However, neurovascular protection has also been seen after systemic infusion of MSCs (Menge et al. 2012), presumably due to a different mechanism . In this setting the tissue inhibitor of matrix metalloproteinase−3 (TIMP−3) has been identified as a critical factor. Iv injected MSCs are mainly trapped in the lungs where, interacting with pulmonary endothelial cells, they secrete TIMP−3 which significantly reduces BBB leakage. Thus, MSCs do sustain the brain vasculature and promote angiogenesis, acting as local bioreactors, as well as interacting with extra-neurologic organs, providing a “remote” systemic control.
11.3 Manipulation of MSCs: Engineered Stem Cells, Survival-Promoting Scaffolds
Tissue engineering combines scaffolds (spatially guiding tissue regeneration), transplantation of cells (engrafting and/or providing a favorable regenerative environment) and delivery of different biomolecules (trophic factors, modulators of inflammatory molecules) in the hope that combinatory strategies may be more effective in achieving tissue repair. Scaffolds are supporting elements of various compositions and shapes. For TBI, which causes a mixture of dead, contused and spared tissue, injectable rather than preassembled scaffolds may be preferred. The infusion of injectable scaffolds minimizes the risk of damaging intact surrounding tissue (Delcroix et al. 2010; Saracino et al. 2013). Collagen (Lu et al. 2007; Qu et al. 2009, 2011; Xiong et al. 2009; Mahmood et al. 2011, 2013b, 2014; Guan et al. 2013), fibrin (Yasuda et al. 2010) and alginate (Heile et al. 2009) scaffolds have been seeded with BM-MSCs and transplanted up to 7 days after injury in rodents. Details of the protective/regenerative effects observed in the different studies, mostly from the same leading group, are provided in Table 11.1. On the whole, implanting human MSCs with scaffolds is more effective than implanting the cells alone. The mechanical and molecular 3D environment of the scaffolds improves transplanted MSCs survival and stimulates their expression of trophic molecules, fostering regenerative processes in the injured brain. Whether these effects depend on the scaffolds increasing delivery of donor MSCs at the injury site, or whether scaffolds also modify the molecular properties of MSCs is not clear. Nanostructured scaffolds for neuroregenerative purposes hold promise, but further work is needed to develop fully biodegradable injectable scaffolds, biocompatible for surgical use.
Table 11.1
Manipulation of MSCs with survival-promoting scaffolds for TBI
Reference | TBI: model/recipient | Scaffolds | Gel state (injectable/preformed) | N° and cell origins | Treatment (timing and location) | Outcome | Mechanisms |
---|---|---|---|---|---|---|---|
Lu et al. 2007 | CCI/rat | Collagen | Preformed (cylinder) | 3×106 human BM-MSCs | 4d post-injury/lesion cavity | Evaluated up to 5 weeks: spatial learning sensorimotor function lesion volume | Cell migration into the lesion boundary zone; neurotrophic support for the surrounding brain tissue |
Xiong et al. 2009 Qu et al. 2011 | CCI/rat | Collagen | Preformed (cylinder) | 3×106 human BM-MSCs | 7d post-injury/lesion cavity | Evaluated up to 5 weeks: spatial learning sensorimotor function neuronal cell loss axonal density | neural fiber length in the injured cortex neurogenesis, angiogenesis and signal transduction tissue plasminogen activator (tPA) and tPA-positive neurons Nogo-A protein |
Qu et al. 2009 | CCI/mouse | Collagen | Preformed (cylinder) | 0.3×106 human BM-MSCs | 7d post-injury/lesion cavity | Evaluated up to 5 weeks: spatial learning lesion volume | vascular density |
Heile et al. 2009 | CCI/rat | Alginate | Preformed (capsule) | 6.4×104human BM-MSCs+ GLP-1 fusion gene transfection | Immediately before-injury ICV | Evaluated up to 2 weeks: neuronal cell loss | GLP-1 level in cerebrospinal fluid |
Guan et al. 2013 | CCI/rat | Collagen | Injectable | 3×106 human BM-MSCs | 7d post-injury/lesion cavity | Evaluated up to 4 weeks: spatial learning sensorimotor function cell survival | Glucose metabolism in the lesion site |
Yasuda et al. 2010 | Cold rod to the right cortex/rat | Fibrin matrix | Injectable | 104mice GFP + BM-MSCs | 7d post-injury/center of the lesion | Evaluated up to 4 weeks: cell survival and migration | GFP + MSC subpopulation expressing-MAP2 and co-localizing with neurons and perivascular cells |
11.4 Challenges Involved in the Therapeutic Use of MSCs
11.4.1 MSC Heterogeneity
The protection observed in experimental models varies widely in different studies. Next to conceptual issues and methodological differences between injury models and laboratories, heterogeneity of MSC populations may contribute to disparate outcomes. This heterogeneity is at various levels. The first is donor-to-donor heterogeneity. Every MSC donor intrinsically differs genetically, physiologically, etc., and this may for example affect the patterns of MSC gene expression, differentiation capacity and secretion of bioactive molecules. Donor age also clearly contributes to differences in BM-derived MSCs, though heterogeneity has also been observed in MSCs isolated from age- and sex-matched donors (Phinney 2012; Dimarino et al. 2013). Another level of heterogeneity is between MSC populations isolated from different human tissues. MSCs from different sources cannot be considered entirely equivalent in terms of their immunophenotype, secretory and proteomic profile, differentiation potential and immunomodulatory ability (Phinney 2012). One explanation may relate to the in vivo origin of MSCs from different tissues, which can influence the commitment, phenotype and functions of the cells differently. However, there are only a few studies directly comparing gene and protein expression and potential of cells isolated from different sources and comparison is further complicated because different laboratories use non-standardized isolation and cultivation methods.
A further level of heterogeneity is intra-population heterogeneity. MSCs isolated from a specific source still tend to be heterogeneous populations which, when cultured, may contain both undifferentiated stem/progenitor cells and more mature cell types, with different functional abilities (Magatti et al. 2008; Bernardo et al. 2009; Meirelles and Nardi 2009). In the case of BM-MSCs this heterogeneity has been detected using different experimental approaches, including transcriptome or immuno-staining analysis as well as assays aimed at investigating differentiation abilities (Phinney 2012). The question of MSC heterogeneity is even more complex since culture and expansion conditions can introduce experimental artefacts, modifying the expression of natively expressed markers and promoting the expression of new ones, altering the original cellular phenotype and functions. Additional work is urgently needed to identify the specific properties of each MSC sub-population and to understand the determinants of intrinsic MSC heterogeneity. This is vital in order to reduce experimental and clinical variability, predict MSC in vivo potency and develop successful MSC-based treatment transferable to the clinic.
11.4.2 Autologous or Allogenic Transplant and Immunosuppression
The choice of rodent or human MSC sources in the experimental regenerative field is still in its early years. On one hand, rodent MSCs allow syngenic or allotransplants which are the conditions faced in the clinical setting (matched human MSCs transplanted in patients). On the other hand, human MSC candidates for clinical use need to be tested in rodent models to assess their efficacy, long-term effects, and safety and to obtain regulatory approvals. The minimum criteria required to define MSCs are applicable specifically to humans, and cannot be entirely extended to cells isolated from other species. Rodent MSCs differ from human MSCs in marker expression and in some of their general characteristics and potency (Peister et al. 2004; Chamberlain et al. 2007) (see Sect. 11.1). If rodent MSCs are fundamental as proof of concept studies, human MSCs offer a higher predictive value but their efficacy and mechanisms of action need to be fully addressed in the experimental setting in order to move to clinical trials .
Autologous stem cells have been the treatment of choice in TBI trials so far. Harvesting patient-specific MSCs poses timing, logistic and standardization constraints. To interact promptly with pathological pathways of secondary damage and to foster restorative processes, MSCs have to be transplanted in the acute phase. This limits the possibility of autologous transplantation. The transplantation of bank-stored GMP-grade certified MSCs may overcome some of the logistic limitations associated with autologous MSCs in the organ transplant setting and allow institutions without GMP facilities or the capacity to isolate MSCs to participate actively in this field of research. While the autologous MSC product can introduce differences in cell potency related to the patient’s age and disease (Pietilä et al. 2012), allogenic cell transplant can be easily standardized and therefore provide more comparable results among different trials (Franquesa et al. 2013). Allotransplant, however, poses the risk of host rejection due to immunological mismatch. Thus, immunosuppression becomes a critical question before cell therapy can move to clinical application.
Data from acute brain injury models, including TBI (Kim et al. 2010; Li et al. 2011; Zanier et al. 2011), stroke (Nomura et al. 2005; Wakabayashi et al. 2010; Xin et al. 2010) and spinal cord injury (Yang et al. 2008; Hu et al. 2010; Cizkova et al. 2011), show the efficacy of allo- and xeno-transplanted MSCs with different immunosuppression strategies (Anderson et al. 2011). MSCs do not appear to retain intrinsic immunogenic properties, do not trigger alloreactivity and can survive and differentiate into allogenic or even xenogenic immunocompetent recipients in vivo (Atoui and Chiu 2012). Thus, MSCs have been proposed as “universal donor cells”. However, emerging reports have challenged the limited immunogenicity of allogenic MSCs (Eliopoulos et al. 2005; Nauta et al. 2006; Knaän-Shanzer 2013) and there are also conflicting findings regarding immunogenicity of differentiated BM-derived MSCs (Knaän-Shanzer 2013). MSCs are rejected after xenotransplantation into the ischemic rodent myocardium and immunosuppression is needed to improve their efficacy and survival in the ischemic heart (Grinnemo et al. 2004, 2006). Transplantation of MSCs into the non-injured adult rodent brain can induce an inflammatory response leading to rapid and complete rejection of the transplanted cells, preventing plastic effects (Coyne et al. 2006, 2007). Consequently, the immunological impunity of MSCs in vivo is not fully supported. Immunosuppression in TBI patients clearly has dangerous implications because it increases susceptibility to infection which is directly related to unfavorable outcomes (Stocchetti 2005; Zygun et al. 2006). We have recently analyzed the specific contribution of immunosuppressive treatment to MSC efficacy for TBI, xenotransplanting human MSCs into mouse hosts, a condition that amplifies immunological mismatch-related problems (Pischiutta et al. 2014). MSCs had similar effects in immunosuppressed and immunocompetent mice on all outcome measurements at 1 month post-TBI (i.e. sensorimotor and cognitive deficits, contusion volume, vascular density, gliotic scar, neurogenesis). No signs of early xenogenic rejection (3 days post-injury) were observed and at longer time points (35 days post-injury). MSCs showed similar presence in immunosuppressed and immunocompetent traumatized brains, indicating that after TBI MSCs injected in the acute phase can escape the normal processes of xenogenic rejection. These results constitute a step forward for the development of MSC therapies for TBI, suggesting that MSCs isolated and expanded from donors, tested for their functional abilities and stored as an “off the shelf” medicinal product, may be made immediately available, with no delay to therapy and multiple doses can be given if necessary.
11.4.3 Cell Administration Route
Various routes of administration of MSCs have been employed in experimental models of TBI and protection has been reported after iv, ia, ic or icv infusion. Thus different routes can be taken into consideration after TBI. Below we discuss some of the advantages and limitations of each route. We also suggest that the biology of TBI and its heterogeneity could be important factors in deciding the route of choice.
11.4.3.1 Systemic MSC Administration
Intravenous Delivery.
A number of preclinical studies (Mahmood et al. 2005; Harting et al. 2009; Zhang et al. 2013) used iv infusion for cell delivery for TBI. The iv route has two important advantages: (1) it is minimally invasive and, compared to direct transplantation into the central nervous system, overcomes the risks of bleeding and tissue injury; (2) it can be done quickly thus allowing timely treatment. However, some limitations also need to be considered. An initial obstacle to iv delivery is the large proportion of first-pass pulmonary sequestration. Many studies in animal models have shown that iv infusion of MSCs does not yield a large number of cells reaching the organ of interest, because the majority are trapped in the lungs (Schrepfer et al. 2007; Fischer et al. 2009). Pulmonary sequestration is primarly related to the MSC size. Schrepfer et al. showed that the mean size of suspended mouse MSCs (15–19 μm) is bigger than the pulmonary capillaries so most of the iv-injected MSCs are trapped in the capillaries, preventing access to the intended organs (Schrepfer et al. 2007). Besides cell size, the expression of adhesion molecule by MSCs is another important factor in pulmonary sequestration. Pre-clinical studies have shown that MSCs interact with endothelial cells, engaging P-selectin and vascular cell adhesion molecule−1 (VCAM−1) (Rüster et al. 2006). The inactivation of VCAM−1 counter ligand (VLA−4/CD49d) on the MSC surface blocks the MSC-endothelial interaction resulting in a significant increase of MSCs in the arterial system (Fischer et al. 2009).
Syngenic stem cell passage across the pulmonary circulation was investigated in anesthetized Sprague-Dawley rats, using silicone tubing catheters placed in the left internal jugular vein and common carotid artery to measure pulmonary passage of MSCs, MAPCs, neural stem cells (NSCs) and BM derived mononuclear cells (BMMCs) (average diameters respectively 18, 15, 16 and 7 µm) co-labeled with specific nanocrystals and infused iv. The labelled cells in the arterial circulation and in peripheral filter organs (lungs, spleen and kidney) were quantified by flow cytometry and infrared imaging, respectively. MSC pulmonary sequestration was 30 times that of BMMCs (Fischer et al. 2009). Two independent studies found that after TBI, only 0.001 % of iv injected cells were found in the brain or organ systems other than lung parenchyma 2–3 days after injection (Harting et al. 2009; Prockop and Oh 2012). These studies strongly suggest that the efficacy after MSC iv infusion is very likely unrelated to the MSCs reaching the injured tissue but that infused MSCs act as remote “bioreactors” stimulating resident cells in lung (macrophages) and spleen (T-cells) to acquire an anti-inflammatory phenotype (Harting et al. 2009; Prockop and Oh 2012; Walker et al. 2012b) thereby promoting resolution of the brain injury.
Intra-Arterial Delivery.
The rationale behind ia administration is to bypass the pulmonary first pass effect, increasing delivery of infused cells to the target tissue. However, microvascular occlusions have been documented and CBF impairment (80–90 % reduction in laser Doppler flow signal) have been shown to occur in 35 % of treated animals (Walczak et al. 2008). More encouraging results were obtained by Lundberg and collaborators (Lundberg et al. 2012) who reported no thromboembolic complications after ia delivery. The presence of human MSCs in the brain was higher compared to iv administration, but the contusion model they used was not associated with any gross neurological symptoms, thus preventing the assessment of stem cell transplantation efficacy.
Additional studies are needed to establish if ia transplantation of MSCs gives more favorable effects than iv injection. Furthermore, while this approach may be particularly interesting in ischemic stroke when an endovascular procedure may already be planned, in TBI patients who have intracranial pressure and perfusion pressure problems, all the complications associated with carotid puncture/manipulation must be carefully evaluated before considering this strategy as promising.
11.4.3.2 Focal MSC Administration
Intracerebroventricular Delivery.
Icv cannulation in human TBI is invasive and may have significant complications; however, it is recommended by authoritative guidelines for intracranial pressure monitoring of severe TBI in the intensive care unit (Brain Trauma Foundation et al. 2007; Stocchetti et al. 2008). In these patients, this site would therefore be free from additional surgical complications and offer the advantage of focal administration directly in the region of interest.
In mice icv administration of human umbilical cord blood or BM-MSC induced lasting improvement in sensorimotor and cognitive functions and reduced contusion volume 1 month after TBI (Zanier et al. 2011; Pischiutta et al. 2014). Our data provide evidence that MSCs can also act as a local “bioreactor” in the brain. In our model icv injected MSCs are detected in the ventricles and at the lesion site for up to 5 weeks in TBI mice, but are confined to the ventricles in sham-operated mice (Pischiutta et al. 2014) supporting a local action of MSCs on host tissue. However, further studies are necessary to see whether if direct contact between MSCs and the injured cells in vivo is needed for protection, as previous evidence suggests that MSCs may act through the release of active molecules rather than through cell-to-cell contact (Zanier et al. 2011; Walker et al. 2012b).
Intracerebral Delivery.
The rationale for ic stem cell implantation is to maximize the MSCs load at the site of injury. However, increasing evidence that MSC engraftment is not required for therapeutic efficacy challenges this approach. Furthermore, BM-MSC differentiation could trigger immune rejection (Niemeyer et al. 2008; Huang et al. 2010) probably due to a switch in surface-MHC molecule composition during MSC differentiation, as described in the heart (Huang et al. 2010). The invasiveness of the ic approach and the possibility of further tissue damage during cell transplantation make this strategy unlikely in the treatment of TBI at the present time.
The decision on the administration route is therefore fundamental in the definition of a clinical protocol. Issues to be considered are the type of injury, the biodistribution of injected cells and the cell type. Iv administration offers easy access and the potential for broad distribution, but has the disadvantage of a large pulmonary first-pass effect, thus significantly reducing the cells delivered to the arterial circulation. Ia delivery can target the injured tissue better, but it can cause emboli, impairing blood flow and worsening the clinical outcome. Icv delivery may be the choice in a selected group of severe TBI patients.
11.4.4 Treatment Timing and Doses
The optimal timing of therapeutic MSC administration after TBI is a point open for discussion. There is ample evidence of the reciprocal interaction between infused MSCs and endogenous cell population. After TBI, the severity and kinetics of the TBI-related metabolic cellular and molecular cascades are the determinants of the injured microenvironment that, with time, may be more or less permissive to MSC functions. At present, technical aspects related to patient stabilization and identification of the patient-matched allogenic MSCs set the lower limit of the window of treatment at approximately 12–24 h post-TBI. No robust data are available to define the upper limit. However, considering the rapid evolution of secondary damage involving pericontusional cerebral tissue that could be rescued, it seems reasonable to treat the patient as soon as possible.
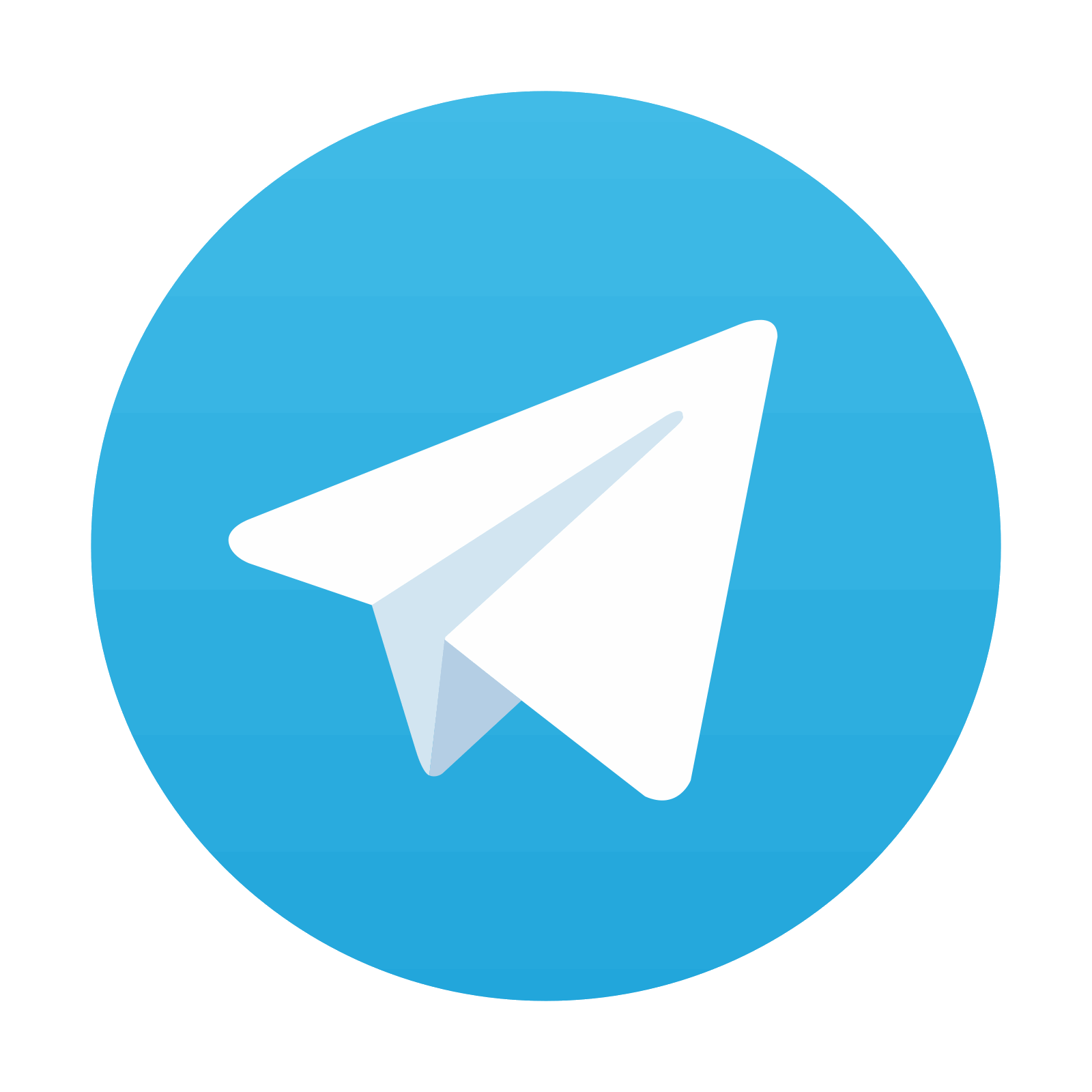
Stay updated, free articles. Join our Telegram channel
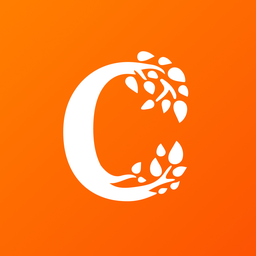
Full access? Get Clinical Tree
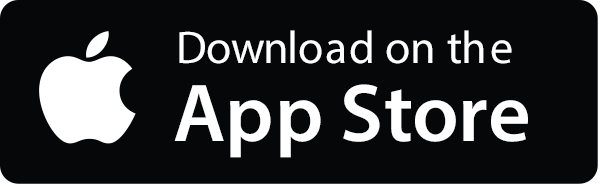
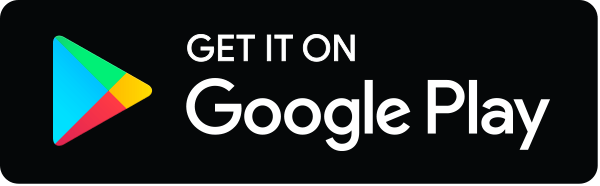