Physical basis of the electrode/electrolyte interface
When a metal electrode is placed inside a physiological medium, such as extracellular fluid (ECF), an interface is formed between the two phases. In the metal electrode phase and in attached electrical circuits, charge is carried by electrons. In the physiological medium or, in more general terms, the electrolyte, charge is carried by ions, including sodium, potassium, and chloride in the ECF. The central process that occurs at the electrode–electrolyte interface is a transduction of charge carriers from electrons in the metal electrode to ions in the electrolyte.
In the simplest system, two electrodes are placed in an electrolyte, and electrical current may pass between the electrodes through the electrolyte. One of the two electrodes is termed a working electrode (WE), and the second is termed a counter electrode (CE). The working electrode is defined as the electrode that one is interested in studying, with the counter electrode being necessary to complete the circuit for charge conduction.
There are two primary mechanisms of charge transfer at the electrode–electrolyte interface, illustrated in Figure 6.1 . One is a non-faradaic reaction, where no electrons are transferred between the electrode and electrolyte. Non-faradaic reactions include redistribution of charged chemical species in the electrolyte. The second mechanism is a faradaic reaction, in which electrons are transferred between the electrode and electrolyte, resulting in reduction or oxidation of chemical species in the electrolyte.

Capacitive/non-faradaic charge transfer
If only non-faradaic redistribution of charge occurs, the electrode/electrolyte interface may be modeled as a simple electrical capacitor called the double layer capacitor C dl . This capacitor is formed due to several physical phenomena . When a metal electrode is placed in an electrolyte, charge redistribution occurs as metal ions in the electrolyte combine with the electrode. This involves a transient transfer of electrons between the two phases, resulting in a plane of charge at the surface of the metal electrode, opposed by a plane of opposite charge, as counterions, in the electrolyte.
If the net charge on the metal electrode is forced to vary (as occurs with charge injection during stimulation), a redistribution of charge occurs in the solution. Suppose that two metal electrodes are immersed in an electrolytic salt solution. Next, a voltage source is applied across the two electrodes so that one electrode is driven to a relatively negative potential and the other to a relatively positive potential. At the interface that is driven negative, the metal electrode has an excess of negative charge (see Fig. 6.1 ). This will attract positive charge (cations) in solution towards the electrode and repel negative charge (anions). In the interfacial region, there will be net electroneutrality, because the negative charge excess on the electrode surface will equal the positive charge in solution near the interface. The bulk solution will also have net electroneutrality. At the second electrode, the opposite processes occur, i.e. the repulsion of anions by the negative electrode is countered by attraction of anions at the positive electrode.
If the total amount of charge delivered is sufficiently small, only charge redistribution occurs, there is no transfer of electrons across the interface, and the interface is well modeled as a simple capacitor. If the polarity of the applied voltage source is then reversed, the direction of current is reversed, the charge redistribution is reversed, and charge that was injected from the electrode into the electrolyte and stored by the capacitor may be recovered.
Faradaic charge transfer and the electrical model of the electrode–electrolyte interface
Charge may also be injected from the electrode to the electrolyte by faradaic processes of reduction and oxidation, whereby electrons are transferred between the two phases. Reduction, which requires the addition of an electron, occurs at the electrode that is driven negative, while oxidation, requiring the removal of an electron, occurs at the electrode that is driven positive. Unlike the capacitive mechanism, faradaic charge injection forms products in solution that cannot be recovered upon reversing the direction of current if the products diffuse away from the electrode. Figure 6.1 b illustrates a simple electrical circuit model of the electrode–electrolyte interface, consisting of two elements . C dl is the double layer capacitance, representing the ability of the electrode to cause charge flow in the electrolyte without electron transfer. Z faradaic is the faradaic impedance, representing the faradaic processes of reduction and oxidation where electron transfer occurs between the electrode and electrolyte. One may generally think of the capacitance as representing charge storage, and the faradaic impedance as representing charge dissipation.
The following are examples of faradaic electrode reactions. Cathodic processes, defined as those where reduction of species in the electrolyte occur as electrons are transferred from the electrode to the electrolyte, include such reactions as:
2 H 2 O + 2 e − → H 2 ↑ + 2 O H − reduction of water
PtO + 2 H + + 2 e − ⇌ Pt + H 2 O oxide formation and reduction
IrO + 2 H + + 2 e − ⇌ Ir + H 2 O oxide formation and reduction
Ir O 2 + 4 H + + 4 e − ⇌ Ir + 2 H 2 O oxide formation and reduction
2 Ir O 2 + 2 H + + 2 e − ⇌ I r 2 O 3 + H 2 O oxide formation and reduction
Pt + H + + e − ⇌ Pt − H hydrogen atom plating
Anodic processes, defined as those where oxidation of species in the electrolyte occur as electrons are transferred to the electrode, include:
2 H 2 O → O 2 ↑ + 4 H + + 4 e − oxidation of water
Pt + 4 C l − → [ PtCl 4 ] 2 − + 2 e − corrosion
Reaction 6.1 is the irreversible reduction of water forming hydrogen gas and hydroxyl ions. The formation of hydroxyl raises the solution pH. Reversible reactions, where species remain bound or close to the electrode surface, are demonstrated by reactions 6.2 through 6.4. Reactions 6.2 and 6.3a, 6.3b, and 6.3c are the reversible formation and subsequent reduction of an oxide layer on platinum and iridium, respectively. Reaction 6.4 is reversible adsorption of hydrogen onto a platinum surface, responsible for the so-called pseudocapacity of platinum. In reaction 6.5, water molecules are irreversibly oxidized, forming oxygen gas and hydrogen ions, thus lowering the pH. Reaction 6.6 is the corrosion of a platinum electrode in a chloride-containing medium.
The electrode interface model of Figure 6.1 b demonstrates the mechanisms of charge injection from an electrode; however it neglects the equilibrium interfacial potential Δφ that exists across the interface at equilibrium. This is modeled as shown in Figure 6.2 a , along with the solution resistance RS (also known as the access resistance RA or the ohmic resistance RΩ) that exists between two electrodes in solution.

If one begins with a system that is in equilibrium and then forces the potential of an electrode away from its equilibrium value, for example by connecting a voltage or current source between the working and counter electrodes, the electrode is said to become polarized. Polarization is measured by the overpotential η, which is the difference between an electrode’s potential and its equilibrium potential (both measured with respect to some third reference electrode):
η ≡ E − Eeq
The net current density across an electrode/electrolyte interface due to a faradaic reaction is proportional to an exponential function of the overpotential, fully described by the current–overpotential equation below .
i net = i 0 { [ O ] ( 0, t ) [ O ] ∞ exp ( – α c nf η ) – [ R ] ( 0, t ) [ R ] ∞ exp ( + ( 1 – α c ) nf η ) }
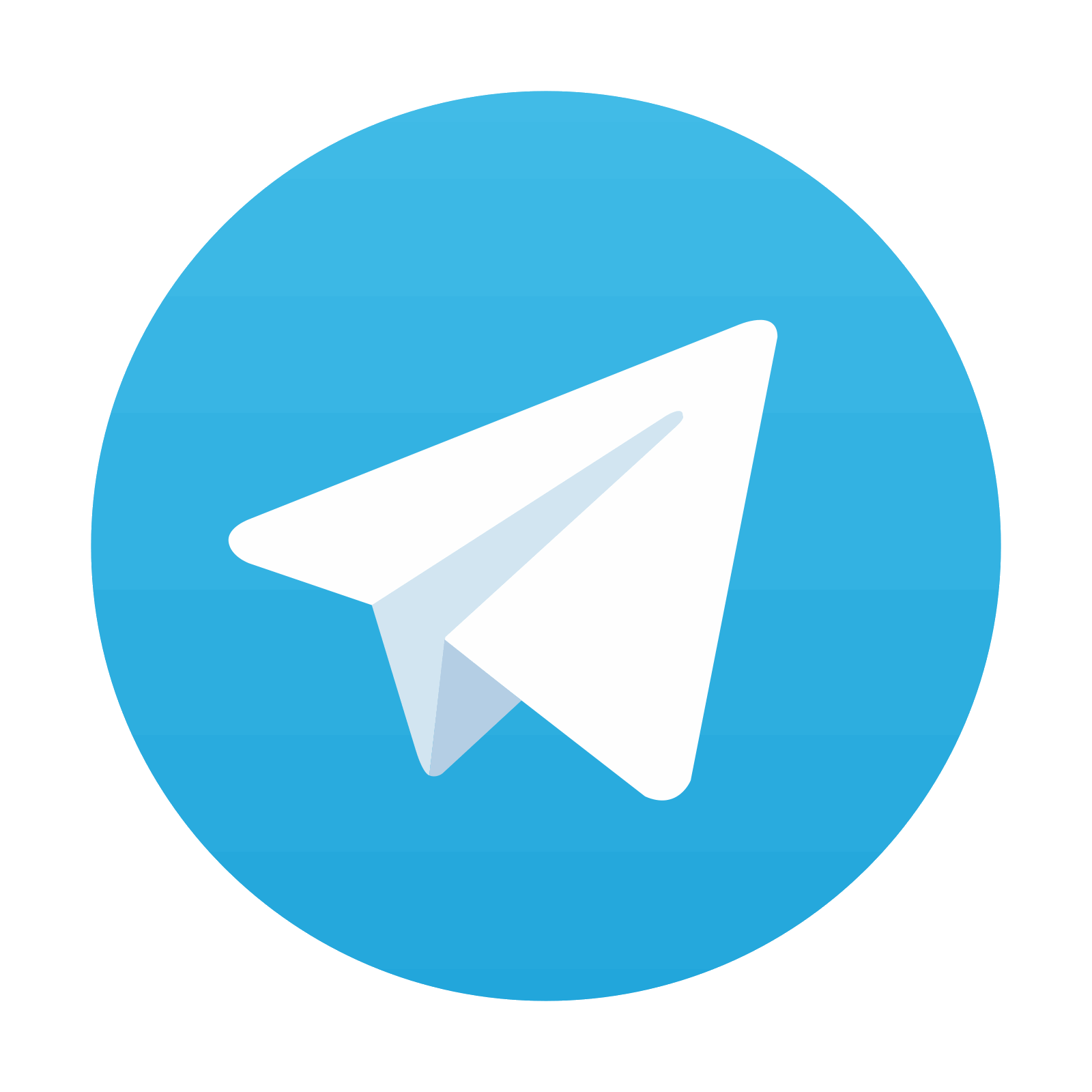
Stay updated, free articles. Join our Telegram channel
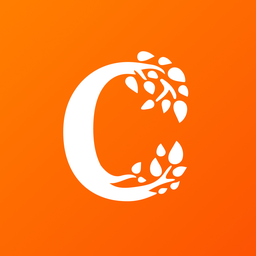
Full access? Get Clinical Tree
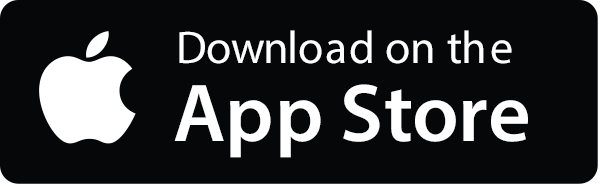
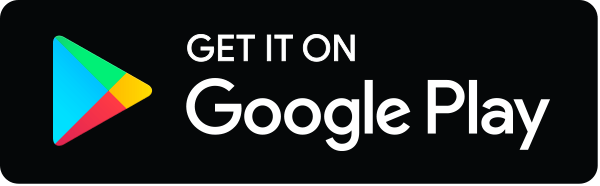