It is an empiric fact that deep brain stimulation (DBS) directly and proximately (antidromicaly and oligosynapticaly) activates wide regions of the brain at time scales comparable to the immediate clinical effect. Further, evidence and reason support the notion that the widespread and simultaneous activations of brain systems are causal to the therapeutic effect of DBS. Combined, these facts and reasoned inferences challenge the most basic tenets of today’s conception of physiology and pathophysiology.
It will be argued that the DBS effects of interest are the result of activating distributed systems, the DBS systems effect hypothesis , rather than specific structures uniquely and in isolation. The DBS systems effect is not the trivial case, in the philosophical sense, that DBS affects wide regions of the brain, eventually. Ultimately, DBS must affect the muscle because motor performance improves, for example in patients with Parkinson’s disease, presumably by engaging the motor cortex (MC), spinal cord and possibly brainstem. The claim of the DBS systems effect hypothesis will be more fully explicated subsequently, but it stands in contrast to claims that the effects of interest, usually those associated with some clinically meaningful effect, are due to actions restricted to the stimulated target; what will be termed the DBS local effect hypothesis . Adjudicating between these conflicting claims first presupposes some notions, implicit or explicit, of causality and how such causality is established. Indeed, it will be argued that the claims of DBS local effect hypothesis were a default position because of the presuppositions current in more general notions of basal ganglia physiology and pathophysiology. Alternatives, such as the DBS systems effect hypothesis, have (had) a more difficult time earning its respect because it opposes not only the DBS local effect hypothesis but also the antecedent notions of causality in basal ganglia physiology and pathophysiology. Thus, the implications of a DBS systems effect hypothesis are wide and deep.
One might argue that the very elegant work by Eidelberg and colleagues demonstrating an effect of DBS on the Parkinson network of neurometabolic changes would argue for a systems effect . However, because of the time scales or temporal resolution of neurometabolic imaging, the network or systems wide effect could be only in the trivial sense as described above. Thus, stimulation of the subthalamic nucleus (STN) or globus pallidus interna (GPi) could result in eventually activating all the nuclei and cortical structures associated with the basal ganglia but at a time scale not relevant to the very rapid dynamics necessary for the effects of DBS or motor control which is over the course of milliseconds. Note, for example, the time difference in the inter-stimulus interval for an effective 130 pulses per second (pps) and an ineffective 100 pps DBS is approximately 3 ms. Yet, this 3 ms difference is sufficient to result in a benefit or none. The issue is raised primarily to demonstrate the importance of understanding the effects of DBS (and basal ganglia physiology in general) on a time scale on the order of milliseconds. Consequently, any analytic or scientific method that does not have a temporal resolution on the order of milliseconds, at best will provide little insight, and at worst, be misleading resulting in complacency.
Evidence in support of a DBS systems effect hypothesis
The following reviews the empiric evidence that DBS activates directly and proximately wide areas of the brain. DBS of the basal ganglia structures will be taken as the exemplar. Note that the argument for the DBS systems effect hypothesis does not discount the observations established by investigators favoring the DBS local effect hypothesis; rather, the criticism is the extrapolation from those observations to inferences of neuronal physiology and pathophysiology sufficient to explain behavior.
First, a definition of what is meant by direct and proximate is offered. Direct implies an effect in a neural element consequent to the electromagnetic fields induced by the DBS pulse. Proximate means that these direct effects have an immediacy, that are operating in a narrow time frame, in this case within one to two synapses from the direct effect. For example, as will be further discussed, the direct effect of the DBS pulse on the efferent axon of the GPi neuron causes an affect in neurons of the ventrolateral thalamus (VL) with the assumption that this affect is important to the clinically important DBS effects. This is to distinguish the proximate effect from the more trivial case of eventual activation of the motor system all the way through to the muscles that is necessary to manifest the clinical DBS effect.
Antidromic activation of axons projecting to and in the vicinity of the DBS
Microelectrode recordings of neurons throughout the basal ganglia–thalamic–cortical (BG–TH–CTX) system during subthalamic nucleus (STN) DBS demonstrate findings consistent with antidromic activation of neurons in the MC, somatosensory cortex (SM), and globus pallidus externa (GPe) . Thus, axons from the MC, SM, and GPe projecting to or in the vicinity of the STN are activated directly by the DBS pulse and that activation is conducted retrograde to cause an action potential in neurons of the MC, SM, and GPe. Figure 8.1 shows peri-event rasters and histograms of neuronal activities following the DBS pulse in the STN of a non-human primate. As can be seen, there is a robust short latency action potential response that is temporally consistent, meaning very little variation in the time of occurrence unlike the more broad and varying subsequent responses. The reason is that most of the variability in communication of information between neurons lies in the diffusion of neurotransmitters across the synaptic cleft which is not a factor in antidromic activation. Further, this response attributed to antidromic activation is seen with different frequencies of DBS stimulation. Regular orthodromic activation requires driving responses in the postsynaptic neuron. Higher frequencies take advantage of temporal summation in the postsynaptic neuron to increase the probability of action potential generation. The robust, short latency, temporally consistent response seen over multiple frequencies are three out of four criteria for an antidromic response. The fourth criterion is collision. To demonstrate collision, one looks for episodes where a spontaneous action potential occurs in the neuron of interest just prior to the DBS pulse. This spontaneous action potential then induces a refractory period that blocks the ascending antidromic action potential and consequently, no response is seen in the neuron.

Unfortunately, in the experiments of which Figure 8.1 is an example, it was not possible to demonstrate collision because insufficient lengths of data were recorded; thereby resulting in rare occurrence of a spontaneous action potential just prior to the DBS pulse. At the time of the experiments, the possibility of antidromic activations was unanticipated.
Antidromic activation of the MC in non-human primates undergoing STN DBS is consistent with the finding of robust cortical electroencephalographic (EEG) potentials at short latencies in response to STN DBS ( Fig. 8.2 ) consistent with antidromic activation. Further evidence of axonal activation came from the EEG evoked potentials in humans using paired-pulse DBS experiments . Pairs of DBS pulses were delivered through the STN and the evoked potentials measured from scalp electrodes. If the time interval between the pair of pulses is more than the refractory period of the stimulated neuronal element, the axon, for example, the effect of the pair of pulses would be relatively doubled compared to a pair of pulses whose interval between pulses were less than the refractory period. There was a marked change in the magnitude of the STN DBS EEG evoked potential between an interval between pulse pairs of 0.5 and 0.75 ms suggesting that the refractory period of the neuronal element responsible for the EEG evoked potential is between 0.5 and 0.7 ms. This refractory period is more consistent with axons than it is with neuronal cell bodies (somas) or dendrites. This is evidence that the EEG evoked potential to STN DBS is mediated by axons and most likely axons between the MC and the STN.

In retrospect, it is understandable that STN DBS would result in antidromic activations of the MC, SC, and GPe as these structures send axons to the STN. Further, biophysical principles demonstrate that axon terminals have the lowest threshold to electrical stimulation.
What could not be anticipated from the anatomy is the antidromic activation of other structures such as the contralateral STN. Microelectrode recordings in the STN contralateral to STN DBS demonstrate clear evidence of increased activity including antidromic activation with collision (unpublished observations). These data clearly demonstrate that axons from the contralateral STN pass close enough in the vicinity of the DBS-stimulated STN to be antidromically activated. The precise terminations of these axons from the contralateral STN running in the vicinity of the STN DBS are unknown.
Alluding to the problematic notion of causation, the question is whether the antidromic activation of the STN neurons contralateral to the DBS are causal to the improvement of symptoms ipsilateral to the STN DBS . If one were to make the reasonable assertion that ipsilateral improvement is related in some manner to neuronal activity changes in the contralateral STN, then the conclusion would be that antidromic activation and increased neuronal activity is related to improvement. At the very least, contralateral STN activation does not worsen ipsilateral symptoms and this contradicts current concepts of basal ganglia pathophysiology where overactivity of the STN is causally related to the bradykinesia of Parkinson’s disease. This will be discussed further subsequently.
Another counterintuitive observation is that GPi DBS produces antidromic activation of VL neurons ( Fig. 8.3 ) Again, axons from the VL pass in the vicinity of the GPi but their precise terminations are unknown. The question arises: how many other axons to and from structures pass in the vicinity of the DBS targets? Current neuroanatomy knowledge is insufficient to answer this question because generally neuroanatomical studies have been interested primarily in the origin and termination of pathways and not the course of the axons. Consequently, there may be many unanticipated and undetected mechanisms evoked by DBS and their causal relations to the clinical effects of DBS are unknown.

Every antidromic activation is associated with corresponding orthodromic activations. For example, antidromic activation of VL neurons in turn causes orthodromic monosynaptic activation of neurons that are the target of VL axons such as the MC and possibly others. Consider antidromic activation of MC neurons. Antidromic action potentials conducted up the MC axon from the STN could invade and be conducted down axon collaterals to many other structures enervated by MC such as other neurons in the ipsi- and contralateral cortex, cerebellum, putamen (PT), STN, red nucleus and others. Thus, there is a myriad of neurons in many structures and systems within one synapse of the STN DBS effect.
Orthodromic activation of neurons remote from the vicinity of the DBS
Figure 8.1 demonstrates robust responses in representative neurons throughout the BG–TH–CTX system in response to STN DBS at latencies and temporal consistencies that would not be consistent with antidromic activations and, consequently, are likely to be orthodromic activations. Further, STN DBS produces orthodromic activation of the contralateral STN . Also, GPi DBS produces orthodromic responses in the basal ganglia receiving neurons of VL . The precise mechanisms underlying the orthodromic activations are not clear. These activations could be a consequence of antidromic action potentials reaching an axon collateral and then orthodromic conduction down the axon collateral to a subsequent neuron. Alternatively, orthodromic action potentials could be initiated in neurons at the axon hillock or first internode in neurons located in the vicinity of the stimulated target.
Evidence related to DBS systems versus local effects hypotheses
The empiric evidence that a great many structures are within oligosynaptic distance of the DBS effect is clear. The issue is whether the widespread effects or the local effects are causal to the clinically meaningful DBS effects. Given that there is some level of organization of structures into systems linked by sequential or parallel monosynaptic connections, some of the widespread effects can be organized into systems effects. For example, the antidromic activation of MC in turn activates the striatum (STR) and STN. The STR in turn activates (inhibition and/or post-inhibition rebound excitation) the GPe and GPi while the STN activates the GPe and GPi. Organization of some of the DBS effects into a set of systems effects does not exclude the incidental activation of axons in passage from systems extraneous to the BG–TH–CTX system. The question now becomes whether activation of a system rather than a specific DBS target nuclei or structure is causal to the clinically meaningful DBS effects.
Establishing cause and effect in any domain is problematic . The large majority of scientific research is based on correlational analyses and it is difficult to distinguish a necessary connection, to use David Hume’s terms from a constant conjunction; hence epiphenomenal. Further, if multiple, different and contrary inferential claims are based on the same observed correlations, it becomes difficult to adjudicate between the conflicting claims. Typically, the decisions required by an adjudicating necessity, such as awarding of grant support, are influenced by non-scientific factors such as which competing school of thought has greater representation or constituency on review panels . There is a great advantage for the incumbent. Consequently, one school of thought may accept the observational correlations established by the contrary school of thought and still dismiss them as epiphenomenal or, more often, just ignore them.
The first key observation is that there are multiple effective targets for DBS for any condition. For example, DBS of the GPi , STN , VL , MC and GPe are effective for the treatment of Parkinson’s disease. The only reason the STR is not included is that STR DBS has not been attempted, to the knowledge of this author*. Added to this list of DBS targets for Parkinson’s disease is spinal cord stimulation as demonstrated in the rodent model of Parkinson’s disease and vestibular nerve stimulation at least for postural stability .
Rationally, there are two options for explanation. First, there are as many different mechanisms as there are effective DBS targets, in the case of Parkinson’s disease there would have to be at least seven different mechanisms; or there is one (or a few) mechanism(s) in common. If one accepts Occam’s Razor, that it is vain to do with more that which can be done with fewer, the reasonable conclusion is that it is likely that there is one (or a few) mechanism(s). This argues for the DBS systems effect hypothesis and against the DBS local effect hypothesis. The key would then be to determine what DBS of GPi, STN, VL, MC, GPe, vestibular nerve and spinal cord (and perhaps STR) have in common.
The observations of multiple effective DBS targets extend to other disorders. For example, DBS of the intralaminar nuclei of the thalamus, GPi, and STN are effective for treatments of dystonia and Tourrette’s syndrome . DBS of the subgenu cingulum and anterior limb of the internal capsule is effective for treating depression . DBS of the anterior limb of the internal capsule and the STN are effective for the treatment of obsessive–compulsive disorder.
The DBS local effect hypothesis only plausibility is its resonance with past and, unfortunately, current theories of basal ganglia physiology and pathophysiology. The GPi Rate theory posits the GPi to be overactive in Parkinson’s disease due to dysinhibition as well as due to increased drive from a dysinhibited STN. There is overwhelming evidence that overactivity of the GPi is not a necessary or sufficient condition to produce parkinsonism . Further, the GPi Rate theory follows from a notion of motor function as a sequential, hierarchical and modular process. The net effect is a perception of the GPi as a sole or dominant actor in basal ganglia physiology and pathophysiology. However, this notion is incorrect and the alternative is to view the basal ganglia as a system or more specifically the BG–TH–CTX system where physiological function is diffusely represented in a parallel and distributed manner throughout the BG–TH–CTX system . Consequently, it makes no sense to talk about the GPi as having a specific or unique function independent of the rest of the BG–TH–CTX system. This illogic is an example of the Mereological fallacy where the properties of a whole are ascribed to a part or parts. Consequently, it makes little sense to attribute the effects of DBS for movement disorders as though they were mediated solely by the GPi.
Regarding evidence for the DBS local effect hypothesis
An extensive review and critique of the evidence offered in support of the DBS local effect hypothesis is beyond the charge of this chapter. However, a few points are warranted. First, any number of experiments confined to the study of a single structure does not and cannot provide evidentiary support for the DBS local effect hypothesis. This is even true when the results appear self-consistent and seem to have external validity according to the supporting theory invoked. Doing so would be falling victim to the Fallacy of Confirming the Consequence, which is of the form ‘if a implies b is true and b is true then a is true; b may be true for any number of reasons other than a such as © and/or d.’ The inference that a is true given the above is only possible to the extent that there is no c or d that also is true or has a reasonable probability of being true. In this case, the argument is ‘if reducing GPi neuronal activity by GPi DBS implies improved parkinsonism and improved parkinsonism is true, then it must be true that reducing GPi neuronal activity by GPi DBS is true’.While reducing GPi neuronal activity by GPi DBS may be true, there is no evidence that this is causally related to the improved parkinsonism because it may be epiphenomenal. Other experiments would be necessary to demonstrate that other mechanisms, particularly those outside of the DBS target, are not causal. Unfortunately, the large majority of research on the neuronal mechanisms have studied only the stimulated target and these are not sufficient to make any claims in favor of the DBS local effect hypothesis, even if they were replicated a million times.
Evidence for the DBS systems effect hypothesis
There are at least two notions of a systems effect. In one case to be argued subsequently is that stimulation of the BG–TH–CTX system ultimately results in activation of the MC and that injecting activity anywhere in the system drives the MC. This would be an argument for a weak (in the philosophical sense of weak and strong) systems effect. It is weak in the sense that it may be possible to improve disorders, such as parkinsonism, by stimulation of the MC alone by injecting a pulse into any structure that sends axons to or received from the MC. A stronger notion of a systems effect is that activation of the MC, while a necessary condition, it is not a sufficient condition. The weak notion of a systems effect would allow activation of only the MC as sufficient. The strong sense implies that activations of other structures within the BG–TH–CTX system are necessary, though not sufficient.
The weak notion of the DBS systems effect hypothesis
STN DBS causes antidromic activation of the MC as described above. GPi DBS antidromically activates VL output neurons, which necessarily cause monosynaptic activation of the MC. Also, it is highly likely that VL DBS also activates MC. It is not known whether GPe DBS also activates directly (via MC projection axons) or indirectly (via activation of VL to MC axons passing near the GPe DBS). This possibility cannot be discounted given the surprising and unanticipated findings of VL activation with GPi DBS. Consequently, one mechanism in common to most, if not all, effective DBS targets for Parkinson’s disease might involve short latency activation of MC. One study in favor of an MC activation as the causal mechanism are the findings of specific activation or inhibition of STN neurons do not reverse parkinsonism in the rodent model. However, activation of MC axons does .
A brief side note, neurometabolic imaging demonstrates reduced cerebral blood flow (though see to the contrary) in regions demonstrating STN EEG evoked potentials and microelectrode recordings demonstrate increased neuronal activities. Thus, there is a disconnect between neurometabolic changes and changes in actual neuronal activity. At the least, there raises serious concerns, and skepticism, in attempting to infer neuronal activities from metabolic changes. Yet, the seductiveness of the neurometabolic images seem to trump direct neurophysiological recordings (see and in reply). One would expect that direct recordings of electrophysiological measures neuronal activities would be a more direct and more meaningful measure.
The strong notion of the DBS systems effect hypothesis
As the effects of DBS on motor function necessarily involve changes in motor unit recruitment, which likely involve changes in MC neuronal behavior, DBS clearly must have an effect on the MC. However, the effect of DBS on MC neurons is very different in the DBS systems effect hypothesis, where the effect is direct (oligosynaptic), compared to the DBS local effect hypothesis (polysynaptic). The weak notion of the DBS systems effect hypothesis posits that the direct activation of the MC, either directly or from anywhere within the BG–TH–CTX system, is both necessary and sufficient.
The strong notion of the DBS systems effect hypothesis is that while direct activation of the MC is necessary, it is not sufficient. Other activities are necessary within the BG–TH–CTX system so that the MC activation becomes sufficient. Further, the response to DBS has to build over time to reach sufficiency. Evidence for this comes from the demonstrations of varying latencies to clinical responses . For example, the reduction of tremor occurs on the order of a few seconds, improvement in bradykinesia in Parkinson’s disease occurs over tens of seconds and changes in gait may take tens of minutes. (Note the very long-term latencies of changes in dystonia and other disorders are beyond the scope of the chapter.) The DBS local effect theory has difficulty accounting for the latencies with the exception of the possibility of neurotransmitter depletion , while other studies discount the significance of any depletion , or the accumulation of other chemical agents that suppress activity such as adenosine . While depletion of neurotransmitters or the accumulation of other agents may take time and hence, explain the latency to clinical effects, it is not at all clear how such changes would explain the differences in the latencies to different clinical effects. The argument would have to be that different levels of depletion and/or accumulations are necessary for the resolution of the different symptoms. However, this risks an unnecessary profusion of causes against which Occam’s admonition, against doing with more that which can be done with fewer, is of good counsel.
The question becomes: is there an evolution of neuronal activity that would correspond to the latencies to clinical effect? Unfortunately, there is a paucity of data and the most appropriate data are from studies not directly intended to examine this question. One would think that further more definitive studies would be considered important and of some priority. One example is the change in VL thalamic neuronal activities in response to GPi DBS shown in Figure 8.3 . There are several features in the post-stimulus raster. There is an initial short latency temporally consistent and robust response indicative of antidromic activation. This is followed by an inhibition beginning at approximately 3 ms and lasting approximately 3 ms probably related to the postsynaptic inhibitory potentials consequent to γ-aminobutyric acid (GABA) release by activated afferents from the GPi. This is followed by a relatively modest increase in VL neuronal activity consistent with post-inhibitory rebound excitability probably mediated by I h conductance channels. There is a much more robust increase in VL neuronal activity following the modest rebound. There are two additional features. It appears that the antidromic activation diminishes over the time course of the DBS train. At the same time, there appears to be a build up of the late robust increase in activity.
There are at least two possible explanations for the decreasing antidromic activation. At the same time as the antidromic action potential is ascending the VL neuron’s axon there is either hyperpolarization or shunting inhibition of the neuronal cell body, perhaps from stimulation of presynaptic elements releasing inhibitory neurotransmitters. However, antidromic activation is very robust as demonstrated by its relative independence on stimulation frequencies. In other words, while high frequency stimulation improves the probability of an orthodromic activation through temporal summation, this is usually not necessary for antidromic activations and, consequently, the probability of a given DBS pulse producing an antidromic activation is relatively independent of stimulation frequency. Also against the possibility of hyperpolarization or shunting inhibition is the relative lack of inhibition just after the DBS pulse and occurring at times when the neuron did not have an antidromic activation of its axon .
Another possibility is the reduction of antidromic activations consequent to an increased probability of ‘collision’. This occurs when there is an orthodromic generation of an action potential in a neuron just as an antidromic activation is initiated in the neuron’s axon. The orthodromic action potential transmitted down the axon is followed by a refractory period in the axon, which prevents the ascending antidromic action potential from reaching the neuronal cell body to manifest a recorded extracellular action potential. The probability of ‘collision’ increases as the discharge rate of the VL neuron increases and particularly if there is a temporal correlation between the generation of the orthodromic action potential generated in the VL neuron and the stimulation pulse producing the antidromic action potential in the axon of the same VL neuron. Thus, the late increase in VL neuronal activity could be the source of the orthodromic action potentials and their refractory periods in the axon that block the ascending antidromic action potentials and prevent their appearance as a recorded extracellular action potential.
Another, albeit indirect, evidence for a temporally evolving neuronal response is seen from the STN EEG evoked potentials (see Fig. 8.2 ). As can be seen, the waveform associated with a single pulse is different in morphology and distribution compared to a brief train of DBS pulses. This suggests that the underlying neuronal responses are different between the two. The interactions between the sequences of pulses in the DBS train affect the evoked potential. These observations warrant further investigation and substantiation.
Resonance effects of DBS
The question then becomes what are the mechanisms underlying the temporal evolution of the DBS response. One possible mechanism would be the progressive depletion of neurotransmitters or accumulation of other agents, however, this is unlikely given the discussion above. An alternative would be resonance effects due to re-entrant activities traversing the BG–TH–CTX system that builds an increasing response . This presupposes the BG–TH–CTX system as interconnected, nested, non-linear, re-entrant polysynaptic oscillators, what is called the Systems Oscillators theory. Evidence for the systems oscillators theory is presented elsewhere .
There are several lines of evidence supporting the Systems Oscillators theory and its extension that DBS causes resonance amplification within the BG–TH–CTX system. Relatively short latency responses would have to be found in most, if not all, of the structures of the BG–TH–CTX system. This has been demonstrated as shown in Figure 8.1 . STN DBS in the non-human primate produces increased neuronal activity in the MC, sensory cortex, PT, GPi, GPe, and VL (not shown) within 6 ms of the DBS pulse. If the DBS effect is to generate activities that repetitively traverse the various oscillators within the BG–TH–CTX system, then one would expect to see increased activity in many, if not all, the various nodes (cortex and nuclei) of the oscillators. This is the case as shown in Figure 8.1 . However, such demonstration is not proof of re-entrant activity.
Some neurons within the BG–TH–CTX system display repetitive behaviors in response to STN DBS in the non-human primate. An example is shown in Figure 8.4 . In the example shown in Figure 8.4 , there are repetitive increases and decreases in the MC neuron’s response to 50 pps STN DBS. In this case, there are three cycles within the 20 ms inter-stimulus interval suggesting a frequency of approximately 150 Hz. This is significant because the Systems Oscillators theory predicts that the fundamental frequency within the VL–MC feedback loop is approximately 147 Hz and further , high frequency DBS at these frequencies is most efficacious for improving upper extremity function in patients with Parkinson’s disease.

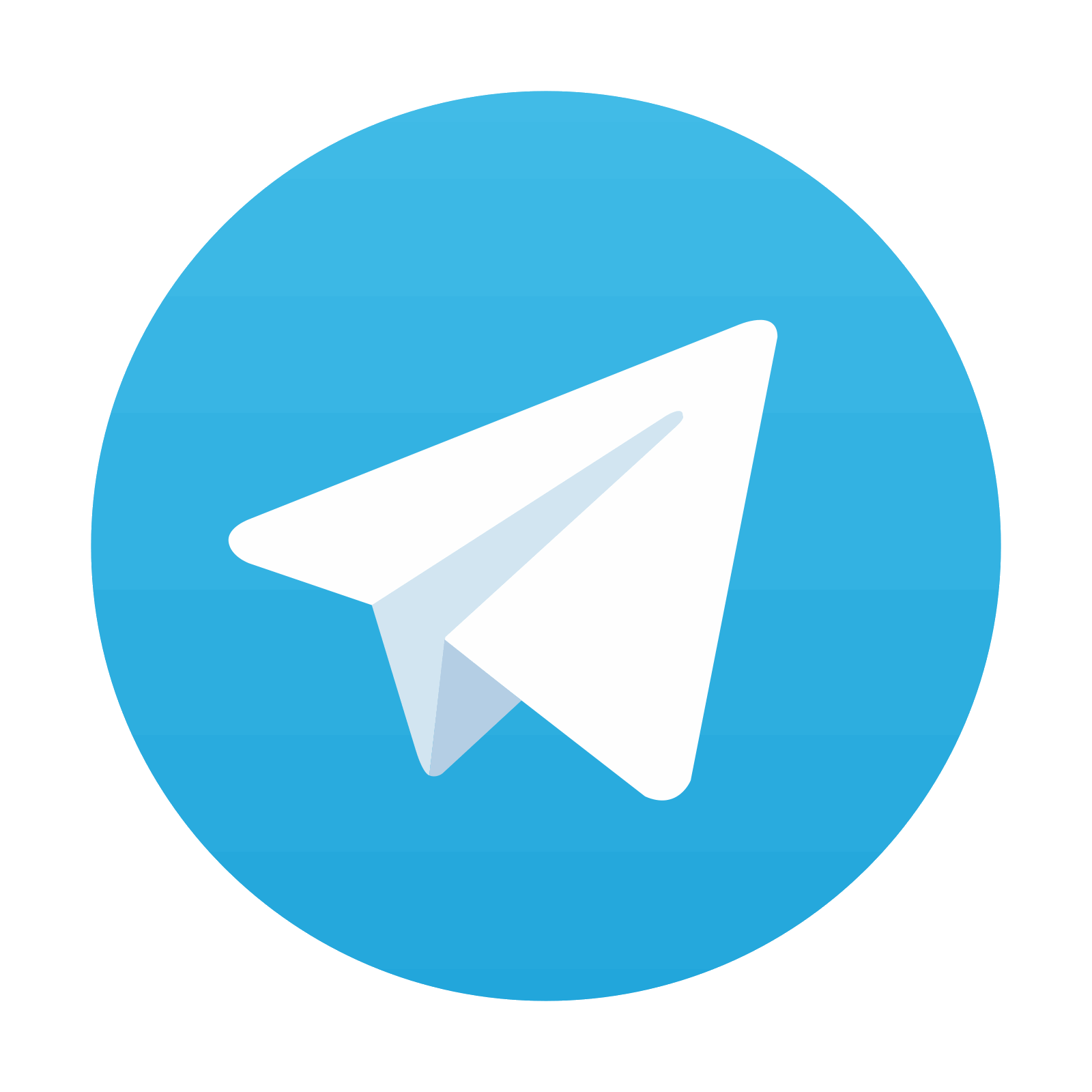
Stay updated, free articles. Join our Telegram channel
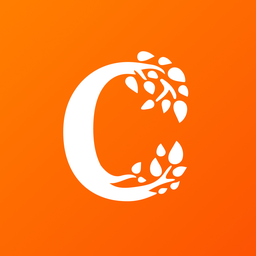
Full access? Get Clinical Tree
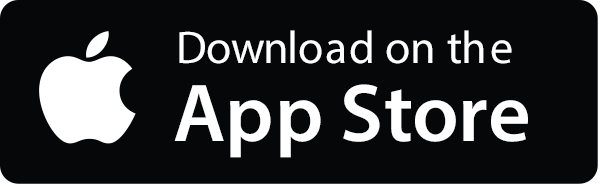
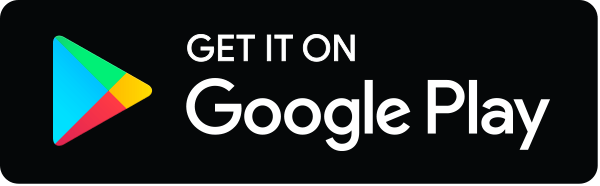
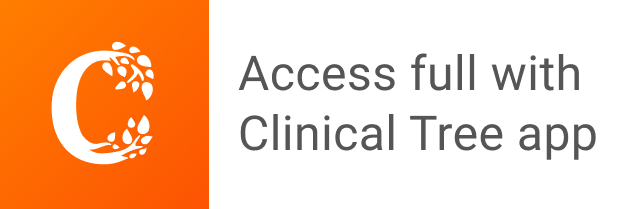