Fig. 17.1
Photolithographic Process. Schematic representation of the photolithographic process used for MEA fabrication

Fig. 17.2
(a) Ceramic Wafer. A ceramic wafer with two different recording site configurations patterned on its surface. Each biomorphic MEA contains 8 recording sites. Recording lines connect each recording site and run the length of the electrode tip for connection to the PCB. The length of each tip is approximately 1 cm. (b) 16-Site MEA. The electrode tip of a 16 site MEA. Photolithographic techniques allow for patterning on the front and back of the ceramic wafer to increase surface recording site density. This photograph shows the Pt recording sites patterned on both sides by using a mirror to reflect the image from the underside of a microelectrode array
After the formation of the MEAs on the ceramic wafer, a diamond saw or laser is used to form or “cut out” the individual MEAs. A major advantage of the diamond saw is that it produces highly polished edges for reduced tissue damage during implantation, but it can lead to small variations in tip sizes. As previously mentioned, the wafer thickness is usually polished to 125 μm. Once cut, the microelectrode tip is ~9,000 μm long and ~1,000 μm at the widest point furthest from the Pt recording sites, where the tip is connected to the printed circuit board (see below). More importantly, the tapered tip of the microelectrode is considerably smaller and varies between 60 and 175 μm. We have found that the tapered design of the MEAs reduces tissue damage surrounding the recording sites.
The ceramic microelectrode tip with Pt recording sites is attached to a printed circuit board (PCB) holder for handling and connection to recording equipment. To connect the ceramic microelectrode to the PCB holder, each bonding pad is wire bonded to an individual Pt recording site on the ceramic microelectrode tip. The tips are epoxied onto the paddle for stability and to insulate the wire bonds. Cutting and assembly is currently performed in conjunction with Hybrid Circuits, Inc., (Sunnyvale, CA). The fully constructed MEAs for anesthetized and freely moving animal recordings are shown in Fig. 17.3a, b, respectively.


Fig. 17.3
Commonly used MEAs. Photographs of the fully fabricated MEAs for anesthetized (a) and freely moving (b) MEAs showing the PCB and wire bonded tip with black epoxy. (c) Magnified images of tips with several Pt recording sites patterned in unique geometrical configurations. The name of the type of MEA tip is shown in the upper right, while the size of the recording sites is shown in the lower left. Where applicable, distance between groups of recording sites is also labeled
17.2.3 Types and Designs of MEAs
As previously mentioned, the major advantage of photolithography is the ability to design MEAs with well-defined, highly reproducible geometrical configurations. Our most commonly used MEAs have four or eight Pt recording sites and are shown in Fig. 17.3c. MEAs are patterned to measure single-unit activity or chemical analytes within layered structures or from multiple brain structures. The R1 provides a larger recording distance that is useful for large brain regions or layered structures, while the S2 and W4 provide dual detection in smaller brain structures. The W3 was designed for simultaneous measurements in the arcuate and median sulci of the non-human primate prefrontal cortex. One of the newest designs is the R2. The 1 mm spacing between pairs of sites makes this microelectrode ideal for measuring multiple brain structures such as the CA1 and CA3 regions of the hippocampus, the prelimbic and infralimbic regions of the prefrontal cortex, and the caudate/putamen and nucleus accumbens of the rat neostriatum. Additional designs are presented in (Gerhardt and Burmeister 2000).
17.2.4 Multiple Uses of MEAs
These biomorphic MEAs have been used for many experiments in both rodents and nonhuman primates and have been applied to chronic recordings of multiple single-unit neural activity, electrical stimulation and in vivo electrochemical recordings of neurotransmitters in awake rats, non-human primates and possibly in future applications to human neurosurgery (Day et al. 2006; Quintero et al. 2007; Dash et al. 2009; Zhang et al. 2009; Konradsson-Geuken et al. 2010; Stephens et al. 2010; Choi et al. 2012; Hampson et al. 2012a, b; Onifer et al. 2012; Opris and Casanova 2014; Howe et al. 2013; Zhou et al. 2013).
These arrays were capable of recording single neuron activity from each of their recording sites for at least 6 months during chronic implantation in the somatosensory cortex of rats (Moxon et al. 2004), and monkey hippocampus (Hampson et al. 2013a) as well as in the prefrontal cortex of nonhuman primates (Opris et al. 2011, 2012a, b). The vertical arrangement of the recording sites on these biomorphic MEAs is ideal for simultaneously recording across the different layers of brain areas such as the cerebral cortex and hippocampus in chronic preparations (Opris et al. 2011, 2012a, b, 2013; Hampson et al. 2012b, 2013a). Hippocampal pyramidal cells in the rat have been stimulated and recorded (Hampson et al. 2013a). Single-unit recordings from nonhuman primate frontal cortex and hippocampus in awake animals have been performed using a specially designed deep recording MEA (not shown) for the primate brain.
Neurochemical recordings of glutamate signaling in the motor and frontal cortex of anesthetized monkeys as well as recordings of glutamate levels in the prefrontal cortex, hippocampus and putamen of awake rodents and nonhuman primates have been performed (Burmeister et al. 2002; Pomerleau et al. 2003; Hascup et al. 2008, 2010; Opris et al. 2012b; Hampson et al. 2013b). New Pt/Ir surfaces formed on the more standard Pt surfaces have been characterized for improved electrical stimulation and enhanced recording site surface area. In conjunction with Ad-Tech® Medical Instrument Corporation, flexible shank electrodes have been developed for future studies in nonhuman primates and patients with epilepsy, brain tumors, Parkinson’s disease deep brains stimulation (DBS) surgery or traumatic brain injury (TBI). In addition, a 4 shank electrode design with 32 active sites for recording and stimulation in the hippocampus of awake nonhuman primates is currently being used and further developed for electrochemical and electrophysiological studies in the hippocampus of awake nonhuman primates. Patterning can also be performed on the reverse side of the ceramic wafer to increase recording site density (Fig. 17.2b).
17.3 Electrophysiological Measures
17.3.1 Signal-to-Noise Ratio of Cell Recordings
Although the acceptable ratio of signal-to-noise is 2:1 for most recordings, the ratios for single and multiple neuron firing recordings with MEAs typically range between 3:1 and 10:1, in both rodent and nonhuman primates (Opris et al. 2011, 2012a, b). For in vivo electrochemical recording, MEA recordings achieve improved signal-to-noise by self-referencing noise subtraction (Burmeister and Gerhardt 2001), but can detect changes in glutamate as low as 0.1 μM/s.
17.3.2 Unit Isolation and Numbers of Units per Pad
The good signal-to-noise ratios of single cell recording achieved by the Pt MEAs allows for very clean isolation of cell waveforms. Extracellular neuron action potentials (spikes) were identified and isolated on-line by separation of extracellular action potential waveform duration and amplitude and confirmed via off-line analysis using principal components analysis and autocorrelation to confirm that (a) single neurons were isolated, (b) all spikes of a given neuron were identified within 95 % confidence limits, and (c) neurons detected simultaneously on adjacent sites were counted only once. In daily recording sessions we were able to isolate up to 4 units per pad (Opris et al. 2011), but 2 units per pad was most common.
17.3.3 Discrimination of Units
To discriminate neurons recorded simultaneously we usually employ a cluster separation method (using Neuroexplorer from www.Plexon.com), such as Principal Component Analysis (PCA) in real time or by offline sorting. We also perform peak-to-peak amplitude separations for cells at each pad where at least two well separated clusters of cells were recorded. All 8 pads discriminated the temporal pattern of neuron spiking from clusters of simultaneously recorded cells (Opris et al. 2011).
17.3.4 Durability and Longevity of the MEA
The durability of MEAs has continuously increased from 3 weeks (Moxon et al. 2004) to up to several years (Deadwyler et al. 2013). A good MEA used in chronic recording can provide reliable units for 6 months to 3 years. In acute recordings the MEAs can be reused many times with very high yield. For example a MEA with 8 pads can provide up to 32 reliable cells per session while a 4 shank MEA with 32 pads can yield up to 128 units in both acute (on a session base) and chronic (on long term base) recordings.
17.3.5 e. Recording Resolution
A good recording resolution comes from the distance between adjacent pads needed for isolation of separate units which is 30 μm. According to anatomic measures by Buxhoeveden and Casanova (2002) and quantitative analysis based on columnar neuron recordings by Mahan and Georgopoulos (2013), this is the ideal separation between pads for biomorphic minicolumnar recordings. Nevertheless, occasionally a cell may fall in between two adjacent pads, and thus one ends up recording the same unit with the adjacent pads. Neurons detected simultaneously on adjacent sites are isolated on only one pad and used for further analysis. On the chemical recording aspect, we have demonstrated that MEAs that are chemically modified for glutamate, acetylcholine, choline, lactate and glucose measures have fast temporal resolution (<1 s), excellent spatial resolution (microns), low detection limits (≤100 nM for all analytes) and cause minimal damage (50–100 μm) to surrounding brain tissue.
17.4 Electrophysiology of Minicolumns with Biomorphic MEAs
Biomorphic ceramic MEAs, designed collaboratively and manufactured in conjunction with Hybrid Circuits and the Center for Microelectrode Technology, University of Kentucky, consisted of eight patterned platinum recording pads on a ceramic substrate (Fig. 17.3c) specifically configured for recording single neuron activity (Hampson et al. 2004; Moxon et al. 2004). The model W3 version of the MEAs (Fig. 17.3c) was specially designed with 20 × 150-μm recording pads 2a–d located to record activity from supragranular (Layer 2/3) cells, whereas pads 5a–d simultaneously recorded neuron activity from infragranular Layer 5 neurons (Fig. 17.4b) in the nonhuman primate. The intra-pad distance between the edges of pads 2a–d and 5a–d was 1,350 μm, with a 30-μm separation between adjacent pads (i.e., 2a–c, 2b–d, etc.). Neural spike trains were analyzed with respect to firing rate during specific events within go/no-go trials (Opris et al. 2011).


Fig. 17.4
Simultaneous Recording of Frontal Cortical Layers and Minicolumns. (a) The recording chamber located in the primate prefrontal cortex. (b) Coronal section in the primate prefrontal cortex with the MEA localized within the upper pad quartet in the supra-granular layers and with the lower pad quartet in the infra-granular layers. (c) The illustration of the MEA recording in prefrontal cortical layers and minicolumns (Adapted with permission from Opris et al. 2011)
17.4.1 Frontal Cortical Cell Activity Recorded with Biomorphic Ceramic MEAs
Neurons (Opris et al. 2011, 2012a, b, 2013) were recorded in Layer 2/3 and Layer 5 and from the dorsal bank of the arcuate sulcus in the dorsolateral prefrontal/premotor areas of prefrontal cortex (PFC). Figure 17.3c shows examples of biomorphic MEA recordings with the W3 MEAs in the dorsal premotor region during go trials of the go/no-go task in nonhuman primates. The waveforms recorded at the vertically separated (1,350 μm) recording sites on the MEA indicate individually isolated neuron firing exhibiting task-related discharges within different cortical cell layers, presumably supragranular Layer 2/3 cells shown at locations 2a–d and infragranular Layer 5 cells shown at MEA site locations 5a–d, 6a–f. Corresponding Post-Event Histograms (PEHs) in Fig. 17.4c illustrate the firing patterns of the same neurons, identified in terms of MEA recording pad location (i.e., 2a, 2b, 5a, 5b, 5a, 6b, etc.) synchronized to target image presentations within the task. The MEA routinely recorded RS (define?) cells from both Layer 2/3 and Layer 5/6 simultaneously in the same session, and as indicated in Fig. 17.4c, most cells showed a significant either increase or decrease (noted by asterisks) in activity related to go/no-go task events.
17.4.2 Layer-Specific Cortical Activity Recorded with Biomorphic MEAs
Implementation of the newly applied MEA technology described here (Burmeister et al. 2000; Hampson et al. 2004; Moxon et al. 2004) allowed simultaneous recording from neurons at two different anatomically and physiologically distinct recording sites in the sensorimotor areas of PFC. The biomorphic design of the MEA allowed identification and designation of activity within a dual laminar arrangement of orthogonal MEA recording sites separated by 1,350 μm, the precise distance between Layer 2/3 and Layer 5 in the PFC Arcuate sulcus (Fig. 17.4a). This recording arrangement allowed unique comparisons of the simultaneous activity of cells from (i) each separate cortical layer and (ii) within the same layer but at adjacent physically separate locations on the MEA (Fig. 17.4b). The differential firing to task-related events of identified PFC cells in both Layer 2/3 and Layer 5 is described in detail in Figs. 17.3–17.5 in terms of individual and mean discharge patterns of simultaneously recorded neurons in each layer during either go or no-go trials.


Fig. 17.5
Glutamate Recordings in Prefrontal Cortex of Awake, Behaving Monkey. (a) MEA for glutamate recording. (b) Glutamate recording with MEA in prefrontal cortical layer 2/3. (c) Electrochemical recording of tonic glutamate neurotransmitter concentrations in PFC Layer 2/3. Mean (±S.E.M.) glutamate concentration ([Glutamate]) measured as a percentage increase over baseline (average 8.69 ± 0.77 μM) glutamate concentration. Horizontal axis indicates phase of DMS task: intertrial interval (ITI), Sample phase, Delay phase (Dly), end of delay phase 5 s prior to Match (PreM), Match phase and reinforcement (Reinf.). Asterisks: *p < 0.01, **p < 0.001, Object vs. Spatial trials; #p < 0.01, ##p < 0.001 DMS task phases vs. ITI. (d) Frequency of phasic glutamate release events measured as transient increase (<2.0 s duration) of at least 5 % in [Glutamate] for the same trials shown E. Frequency normalized to # events per second per DMS trial (Adapted with permission from Opris et al. 2012b)
Our recent results (Opris et al. 2011, 2012a, b, 2013) demonstrate many of the functional “operations” described above in which PFC cortical neurons in both the supragranular and the infragranular layers differentially encoded sensory stimuli relevant to performance of a simple go/no-go task. In addition, firing to task-related events was shown to be encoded between layers by synchronized firing of cell pairs comprising apparent functional “minicolumns” connecting the supragranular and the infragranular layers. This assessment was made possible by use of biomorphic MEAs designed to sample neuronal activity in a manner that would reveal the demonstrated functional columnar organization of the PFC. The results provide new insight into the manner in which underlying PFC microcircuitry integrates convergent sensory, cognitive and motor signals from other brain regions to select and control task-related behavioral response tendencies in primate brain (Opris et al. 2011, 2013).
17.5 Neurochemistry of Cortical Layers and Minicolumns
17.5.1 Amperometry
Amperometric measurements of L-glutamate using biomorphic MEAs provide spatially and temporally resolved measures of neuromolecules in the central nervous system of rats, mice and non-human primates (Talauliker et al. 2011). Although the functional capabilities of MEAs have been previously documented for both anesthetized and freely-moving paradigms, the performance enabling intrinsic physical properties of the MEA device have not heretofore been presented. In these studies, spectral analysis confirmed that the MEA recording sites were primarily composed of elemental platinum (Pt°). In keeping with the precision of the photolithographic process, scanning electron microscopy revealed that the Pt recording sites have unique microwell geometries postfabrication. Atomic force microscopy demonstrated that the recording surfaces have nanoscale irregularities in the form of elevations and depressions, which contribute to increased current per unit area that exceeds previously reported microelectrode designs. The ceramic substrate on the back face of the MEA was characterized by low nanoscale texture and the ceramic sides consisted of an extended network of ridges and cavities. Thus, individual recording sites have a unique Pt° composition and surface profile that has not been previously observed for Pt-based MEAs. These features likely impact the physical chemistry of the device, which may influence adhesion of biological molecules and tissue as well as electrochemical recording. This is likely contributing as well to the unique electrophysiological recording capabilities of the MEAs for single and multi-unit neuronal activity (see above).
17.5.2 Glutamate Recording
17.5.2.1 In Rodents
A major issue that has surrounded in vivo measures of glutamate has surrounded the origin of the signal (see (Timmerman and Westerink 1997). Both neuronal and glial processes are involved in the regulation of tonic glutamate levels and many prior microdialysis studies support that resting levels sampled by microdialysis methods may not be derived from neurons. Recent pharmacological studies in awake rats using glutamate MEAs have shed light on the origins of tonic and phasic glutamate signals (Rutherford et al. 2007; Hascup et al. 2010). Modulation of resting glutamate levels in rat frontal cortex is shown in Fig. 17.5.
Resting glutamate levels were significantly lower (~40–50 %) than vehicle with local application of the Na+ channel blocker TTX or the Ca2+ channel blocker ω-conotoxin. These results support that the resting levels of glutamate measured by the MEAs in the rat frontal cortex are derived from neurons because the inhibitory effects of both a sodium channel blocker and a calcium channel blocker are known to directly affect the release of neurotransmitters from neurons. Second, the contribution of Group II metabotropic glutamate receptors (mGluR2/3), which act as inhibitory auto-receptors and reside primarily on presynaptic neurons in the rat frontal cortex, was examined. An ~35 % increase in resting levels was observed after delivery of the mGluR2/3 antagonist LY341495 while an ~20 % decrease was found with the mGluR2/3 agonist LY379268. These results support the idea that there is a significant neuronal glutamate contribution to resting levels as well as due to the modulation of resting levels detected following local administration of these drugs that can directly affect output of glutamate from nerve terminals. Third, when the non-selective glutamate transporter inhibitor D,L-threo-β-benzyloxyaspartate (TBOA) was locally applied there was an ~120 % increase in resting levels, supporting the idea that extracellular glutamate levels are regulated by glutamate transporters located largely on glia (see Danbolt 2001) and indicating that there is significant spontaneous efflux of glutamate that is detected following glutamate uptake inhibition by the NEAs in vivo.
17.5.2.2 In Nonhuman Primates
Biomorphic ceramic MEAs utilized in prior recordings of nonhuman primate PFC cortical layer 2/3 and layer 5 neurons were used in this study to record tonic glutamate levels and transient release in layer 2/3 PFC (Hampson et al. 2013b). Tonic glutamate levels were seen to increase in the Match (decision) phase of a visual delayed-match-to-sample (DMS) task, while increased transient glutamate release occurred in the Sample (encoding) phase of the task. Further, spatial vs. object-oriented DMS trials evoked differential changes in glutamate levels. Thus, the same biomorphic MEAs were capable of electrophysiological and in vivo electrochemical recording, and revealed similar evidence of neural processing in layers 2/3 and layer 5 during cognitive processing in a behavioral task in awake nonhuman primates.
17.6 Future Developments of MEAs
17.6.1 Measuring Multiple Neurotransmitters Simultaneously
A major advantage of microdialysis is the ability to measure multiple neurotransmitters simultaneously (assuming good separation of peaks during offline detection by high performance liquid chromatographic-based methods). Our laboratory has successfully demonstrated the ability to measure acetylcholine and choline simultaneously (Burmeister et al. 2008), which is an essential element of the enzymes involved. Ideally, the long-term goal is to design an MEA to measure multiple neurotransmitters from the same brain structure. As previously mentioned, photolithographic methods allow us to design MEAs with well-defined, highly reproducible geometric configurations that can be patterned onto the front and back of a ceramic wafer. By using both sides of the ceramic wafer we can greatly enhance the recording site density of an MEA. We have designed several 16 site MEAs, one of which is shown in Fig. 17.2b. By increasing the recording site density we can selectively coat recording sites for self-referencing measurements of different analytes in the same brain region.
17.6.2 Simultaneous Electrophysiological and Electrochemical Recordings
The ability to simultaneously measure local field potentials (LFPs) and neurochemical dynamics would greatly enhance our understanding of neuromodulation. This would have profound implications for studying behavior or disorder related changes in the CNS. Until recently, few studies were capable of achieving simultaneous electrophysiological and electrochemical measures from the same recording preparation. And, those that did either switched between neuronal spike recording and the in vivo electrochemical recordings (Williams and Millar 1990; Stamford et al. 1993; Cheer et al. 2005) or required the use of two separate recording systems (Zhang et al. 2009, 2010). This made the experimental setups cumbersome and expensive while making data analysis and interpretation difficult. However, Zhang and colleagues (2009, 2010) are the first to demonstrate simultaneous LFPs and local neurochemical measurements using a single MEA coupled with constant potential amperometry. They successfully demonstrated that the high frequency component of the amperometric signal resembled LFPs. Using a single set-up and biosensor for the simultaneous acquisition of electrophysiological and neurochemical information can greatly enhance the field of in vivo electrochemistry (Zhang et al. 2009, 2010).
17.6.3 Optogenetics
Due to great diversity of cell types in neural circuits, it is critical to be able to analyze how these different kinds of cells work together. An emerging approach with broad implications for basic and clinical neuroscience is based on optogenetic stimulation (Gradinaru et al. 2007; Tye and Deisseroth 2012). Recent developments in optogenetics based on optical manipulation of activity in neural circuits with light-sensitive rhodopsins, such as the Chlamydomonas channelrhodopsin-2 (ChR2) are now capable to illuminate the inter-laminar microcircuits at the millisecond-scale, with cell type-specific optical perturbations in nonhuman primates (Diester et al. 2011; Han 2012), opening up new possibilities for repair and augmentation. In recent years, MEAs with integrated optical fibers use light-assisted perturbation and recording of local neural circuits during animal behavior (Royer et al. 2010; Marshel and Deisseroth 2013; Bernsterin and Boyden 2011).
17.6.4 Prosthetics
This aspect will be described in another chapter, here we just briefly touch on the relevance of MEAs in neural prosthetics.
17.6.4.1 Decision-Making in PFC
The cognitive approach to neural prostheses holds the promise of assisting individuals who are unable to move but who are capable of making decisions and movement plans. An executive prosthesis may even correct in real time a decision making process before a failed choice. A decision circuit is defined as a closed neural network that measures the probable value of a signal element and makes an output signal based on the value of the input signal and a predetermined criterion or threshold (Ratclif et al. 2003). The biomorphic MEAs provide the basis for applying a system specific model to control firing of cells via application of electrical stimulation (Opris et al. 2011, 2012a, b; Hampson et al. 2012a) to the same loci in which columnar firing has been detected (Opris et al. 2012b; Hampson et al. 2012a). This same model was implemented, tested and demonstrated to facilitate performance (Hampson et al. 2012a, b). This might very well serve as a blue print for a decision chip (Opris 2013).
17.6.4.2 Memory in Hippocampus
Berger and colleagues (Berger et al. 2007, 2008, 2011, 2012) demonstrated in rodents for the first time that a neural prosthesis is capable of real-time identification and manipulation of the encoding process that can restore and even enhance cognitive mnemonic processes. The idea was to block the ability to form long-term memories by using pharmacological agents that disrupt the neural circuitry between two fields of the hippocampus, CA1 and CA3, which interact to create long-term memory. By employing an artificial hippocampal system based on the multiple-input, multiple-output (MIMO) model (Song et al. 2009; Kim et al. 2006) they could duplicate the pattern of interaction between CA3-CA1 by monitoring the neural spikes in cells recorded by the electrode array, and then playing back the same pattern on the same array. Long-term memory capability returned to the pharmacologically blocked rats following activation of the electronic device, programmed to duplicate the memory-encoding function for that specific memory (which lever to pull). This suggests that if a prosthetic device and its associated electrodes were implanted in animals with a normal, functioning hippocampus, the device could actually be used to strengthen the memory being generated internally in the brain and enhance the memory capability of normal animals.
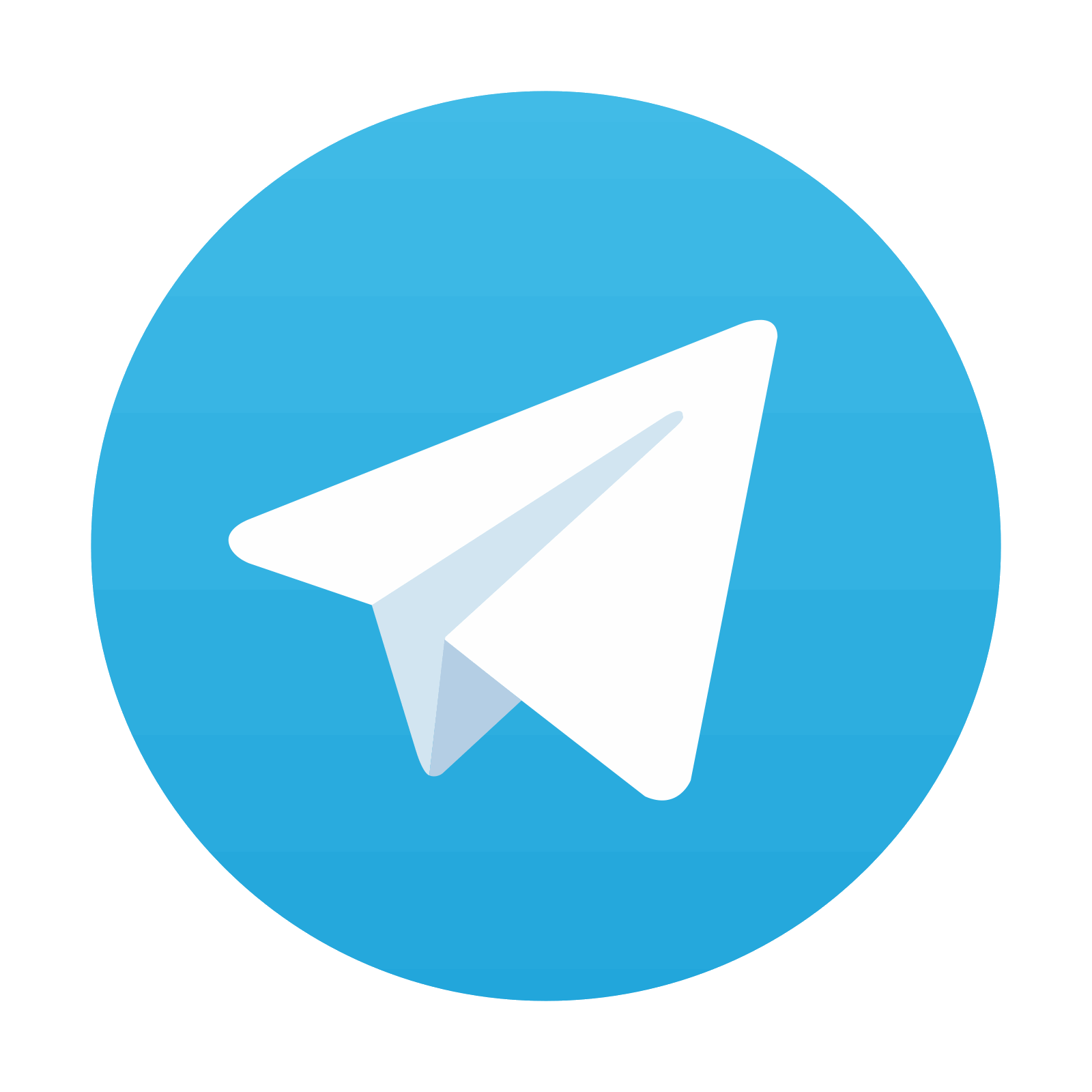
Stay updated, free articles. Join our Telegram channel
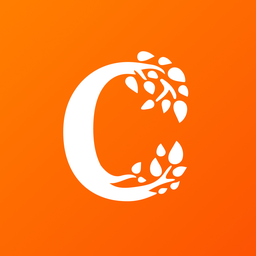
Full access? Get Clinical Tree
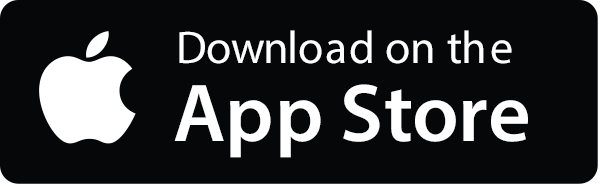
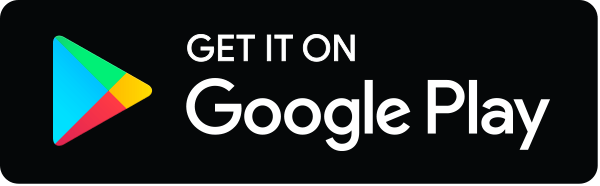