Figure 13.1 A process-based model for explaining working memory processes in the dorsolateral prefrontal cortex.
Based on these findings, we proposed a process-based model to understand how working memory is performed in the DLPFC (Funahashi 2001). As shown in Figure 13.1, this model includes four neural processes: a selection process, a temporary information-storage process, an output process and modulatory inputs. Since the DLPFC is included in the multimodal association areas (Mesulam 2000), the DLPFC receives a variety of information including sensory, motor, motivational, and emotional information from other cortical and subcortical areas. In addition, in order for the DLPFC to perform such higher cognitive functions as thinking, reasoning, decision-making and language comprehension, the DLPFC not only receives a variety of information from cortical and subcortical areas but also sends a variety of information to those areas. Although the DLPFC receives a variety of information, the information temporarily maintained and processed in the DLPFC would be the information necessary to achieve a current goal. To maintain and process the necessary information to achieve a current goal, a neural process for selecting essential information among a variety of information (selection process) would be required. The temporary information-storage process is another essential component of the neural mechanism of working memory in the DLPFC. This neural process would temporarily maintain the information which is selected by the selection process. In addition, by interacting among temporary information-storage processes and other processes, maintained information would be processed, replaced and updated. Therefore, interactions among temporary information-storage processes and other processes can be considered a mechanism for dynamic information processing and a mechanism (p.216) for creating new information. The output process would send out maintained information to the brain areas where the information is utilized. Target areas of the output process would be the areas related to motor outputs, the areas related to long-term memory, and the areas where the DLPFC is monitoring their operation. The modulatory input is a signal or information to modulate the information maintained in the temporary information-storage processes. The modulatory input can be created within the DLPFC or sent to the DLPFC from other brain areas. The modulatory input includes feedback information from motor centers, motivational or emotional information from the limbic areas (Barbas 1992), or modulatory signals by catecholaminergic or monoaminergic inputs (Arnsten 1998; Sawaguchi 1998; Sawaguchi and Goldman-Rakic 1994; Wang et al. 2004; Williams and Goldman-Rakic 1995).
In this model, we hypothesized dynamic and flexible modulation in the strength of functional interactions among different processes and among temporary information-storage processes. Dynamic and flexible modulation in the strength of functional interactions between neurons has been observed in the DLPFC depending on the context of the behavioral task (Funahashi 2001; Vaadia et al. 1995). In addition to the interactions among temporary information-storage processes, modulatory inputs sent from other brain areas could also play a significant role in modifying or updating the information maintained in temporary information-storage processes. Thus, information processing in working memory could be explained as a change of the information represented in temporary information-storage processes caused by dynamic interactions among these processes.
Temporary information-storage process
Delay-period activity, most of which is tonic sustained activation observed during an imposed delay period, has been considered to be a neural correlate of temporary information-storage processes (Funahashi 2001; Funahashi and Kubota 1994; Funahashi and Takeda 2002; Fuster 1997; Goldman-Rakic 1987; Miller 2000). Neurophysiological studies using spatial working memory tasks such as the delayed-response task have revealed that many neurons in the DLPFC exhibit delay-period activity (Funahashi et al. 1989; Fuster 1973; Kojima and Goldman-Rakic 1982; Niki 1974; Niki and Watanabe 1976). The duration of delay-period activity is prolonged or shortened depending on the length of the delay period (Funahashi et al. 1989; Fuster 1973; Kojima and Goldman-Rakic 1982). This activity is observed only when monkeys perform correct behavioral responses (Funahashi et al. 1989; Fuster 1973). If the monkey made an error, delay-period activity was truncated or not observed. A great majority of delay-period activity exhibits a directional or positional preference (Funahashi et al. 1989), such that delay-period activity was observed only when a visual cue was presented at a particular area in the visual field. Many DLPFC neurons exhibited directional delay-period activity, and preferred directions of this activity differed from neuron to neuron. Based on these observations, it has been proposed that neurons having directional delay-period activity have mnemonic receptive fields (memory fields) regarding visual cues in the visual field (Funahashi et al. 1989; Rainer et al. 1998). In addition, using delayed pro- and antisaccade tasks, it has been shown that the great majority (about 70 per cent) of delay-period activity represented information regarding the position of the visual cue, whereas the minority represented information regarding the direction of the saccade (Funahashi et al. 1993). Similar results have been observed by Niki and Watanabe (1976) using a manual delayed-response task and a conditional position task and by Takeda and Funahashi (2002) using conventional and rotatory versions of the oculomotor delayed-response (ODR) task. Thus, delay-period activity represents either retrospective or prospective information, although the majority of delay-period activity represents retrospective information in the DLPFC.
(p.217) Experiments using nonspatial working memory tasks (e.g., delayed matching-to-sample tasks and delayed conditional tasks) have revealed that delay-period activity also represents the active retention of nonspatial information, including faces (O’Scalaidhe et al. 1997; Wilson et al. 1993), object shapes, patterns, or colors (Asaad et al. 1998; Freedman et al. 2002; Miller et al. 1996; Rainer et al. 1999; Rao et al. 1997; Sakagami and Niki 1994). Romo et al. (1999) showed that the magnitude of delay-period activity varied as a monotonic function of the base stimulus frequency in a somatosensory discrimination task, in which monkeys were required to discriminate the difference of frequency between two mechanical vibrations (the base stimulus and the test stimulus) applied to the fingertips. Based on this result, Romo et al. (1999) concluded that this monotonic stimulus encoding may be a fundamental representation of one-dimensional sensory stimulus quality in working memory. In addition, White and Wise (1999) found rule-dependent neuronal activity in the DLPFC. They reported that one-third to one half of task-related neurons showed statistically significant differences in task-related activity depending on the task rule (either a conditional or a spatial rule), indicating that these neurons retained rules of behavioral tasks by modulating the magnitude of task-related activity. Similar rule-dependent activity has also been reported by Wallis et al. (2001). Further, it has been shown that delay-period activity represents reward information (Hikosaka and Watanabe 2000; Kobayashi et al. 2002; Wallis and Miller 2003; Watanabe 1996).
Thus, delay-period activity observed in the DLPFC can be considered a neural correlate of the temporary information-storage process (Funahashi 2001; Funahashi and Kubota 1994; Funahashi and Takeda 2002; Fuster 1997; Goldman-Rakic 1998; Miller 2000). A wide variety of information is temporarily maintained in this process, including visuospatial information, nonspatial visual features, quality and quantity differences in one stimulus modality, motor outputs, or task rules. Regional differences have been proposed in processing information. For example, Goldman-Rakic proposed that the mid-dorsolateral sector mainly participates in visuospatial information processing, whereas the mid-ventrolateral sector mainly participates in information processing of nonspatial visual features (Wilson et al. 1993; O’Scalaidhe et al. 1997; Goldman-Rakic 1998). Hoshi (2006) also suggests that at least two distinct subregions (dorsal sector and ventral sector) can be identified based on region-specific neural activity within the DLPFC. However, neurons having various task-related activities and various spatial and nonspatial features of task-related activity were widely distributed within the DLPFC with substantial overlap (Carlson et al. 1997; Quintana and Fuster 1999; Rainer et al. 1999; Sakagami and Tsutsui 1999). A number of neurons exhibited task-related activity in both spatial and nonspatial working memory tasks (Rao et al. 1997). These observations indicate that neurons in the DLPFC can maintain a variety of information, although each neuron’s preferred information seems to be different from neuron to neuron, and that neurons representing different information are intermingled in the DLPFC.
Information processing
Information processing in working memory could be described as a change of the information represented in temporary information-storage processes caused by dynamic interactions among these and other processes. Neurophysiological studies have revealed evidence for manipulating or integrating information by interactions among processes in the DLPFC. One example is observed in the interaction between neurons having delay-period activity and neurons having post-saccadic activity. It has been shown that many DLPFC neurons exhibit saccade-related activity when saccadic eye movements are used as behavioral responses (Boch and Goldberg 1989, Funahashi et al. 1991; Takeda and Funahashi 2002). Most of saccade-related activity was post-saccadic in the DLPFC (Funahashi et al. 1991; Takeda and Funahashi 2002). Since post-saccadic activity started after the (p.218) initiation of the saccadic eye movement, this activity is not directly related to the initiation as well as the control of the eye movement. However, most of this activity exhibited directional selectivity, such that post-saccadic activity was observed only when the monkey made a saccade toward a particular direction. Therefore, post-saccadic activity can be considered as feedback signals from oculomotor centers which directly participate in the initiation and the control of the eye movement (Funahashi et al. 1991). On the other hand, it has been shown that delay-period activity terminated soon after the subject performed a response behavior. A comparison of temporal profiles between the initiation of post-saccadic activity and the termination of delay-period activity revealed that the termination of delay-period activity coincided with the initiation of post-saccadic activity, suggesting that post-saccadic activity controls the timing of the termination of delay-period activity (Funahashi and Kubota 1994; Funahashi and Takeda 2002; Goldman-Rakic et al. 1990). Erasing unnecessary information from the temporary information-storage process is an important action of working memory. Therefore, post-saccadic activity or feedback inputs from motor centers could serve as a signal to erase unnecessary information from the temporary information-storage process by terminating delay-period activity.
Further evidence for processing and integrating information by interactions among neurons having delay-period activity has been shown by experiments using delayed-response tasks with sequential movements (Funahashi et al. 1997; Inoue and Funahashi 2002). These studies showed that delay-period activity represented composite information when monkeys performed delayed-response tasks combined with sequential hand-reaching behavior (Funahashi et al. 1997) or sequential saccades (Barone and Joseph 1989; Inoue and Funahashi 2002). These include delay-period activity representing a pair of different spatial positions, delay-period activity representing the temporal order of the presentation of multiple cues, and delay-period activity representing a pair of different spatial positions of the visual cues as well as the temporal order of their presentation (Barone and Joseph 1989; Funahashi et al. 1997; Inoue and Funahashi 2002). When activities were recorded during a conventional delayed-response task in which a single spatial cue was presented in each trial, most of these neurons exhibited delay-period activity with simple and monotonic directional selectivity (Funahashi et al. 1997; Inoue and Funahashi 2002). Therefore, delay-period activity representing composite information might be created by interactions among neighboring neurons representing different spatial features.
Several studies have shown that the information represented by prefrontal activity changes with the progress of the task. For example, using a delayed paired association task, Rainer et al. (1999) showed that although prefrontal activity primarily represented characteristics of the sample stimuli (sensory-related coding) during the early phase of the delay period, this activity began to represent characteristics of anticipated targets (prospective coding) toward the end of the delay period. Similarly, using a spatial delayed matching-to-sample task, Sawaguchi and Yamane (1999) showed that spatial information was tuned broadly by delay-period activity in the early phase of the delay period. However, the proportion of neurons exhibiting sharper spatial tuning and high spatial discriminability increased in the later phase of the delay period. Further, Asaad et al. (1998) showed that neural activity conveyed the direction of the impending eye movement progressively earlier along successive trials while monkeys performed arbitrary cue-response association tasks. Quintana and Fuster (1999) observed DLPFC neurons attuned to the cue color and neurons attuned to response directions while monkeys performed working memory tasks using color cues. They found that the discharge of neurons attuned to the cue color gradually diminished during the delay period, whereas the discharge of neurons attuned to response directions gradually increased. These results indicate that the modulation of the temporal pattern of neural discharges reflects the change of the information represented by the neuron and that the information represented by the temporal pattern of the firing of a neuron or an assembly of neurons alters gradually as the trial progresses.
(p.219) Takeda and Funahashi (2002) examined DLPFC activity using two kinds of oculomotor delayed-response tasks (ODR and R-ODR tasks). In the ODR task, the monkey was required to make a saccade to the direction where the visual cue was presented, whereas in the R-ODR task, the monkey was required to make a saccade 90° clockwise from the direction where the visual cue was presented. They showed that the majority of delay-period activity represented the direction of the visual cue, whereas the minority of delay-period activity and the majority of response-period activity represented the direction of the saccade. These results suggest that the transformation from visuospatial information to motor information is carried out in the DLPFC. Since both visuospatial information and motor information can be depicted as directional preference of neural activity, transformation from visual information to motor information could be demonstrated as the temporal change of the preferred direction of neural activity along trials of ODR and R-ODR tasks. Takeda and Funahashi (2004) used population vectors to demonstrate the temporal change of the preferred direction of a population of DLPFC activities with the progress of a trial of the ODR task and the R-ODR task. Figure 13.2a shows population vectors calculated by a population of DLPFC activities at the 180° trial of the ODR task. Population vectors were mostly directed toward the 180° direction. Since the direction of the visual cue and the direction of the saccade were the same in the ODR task, this result indicates that the same directional information is maintained along the delay period (see Figure 13.2c). Figure 13.2bshows

Figure 13.2 (a) Temporal change of the directions of population vectors along the 180° trial of the ODR task. Most of population vectors were directed toward the 180° direction. (b) Temporal change of the directions of population vectors along the 180° trial of the R-ODR task. The direction of the population vector gradually rotated from the 180° direction to the 90°; direction during the delay period. (c) The difference between the vector direction and the cue direction along the ODR trial. The population vector was directed toward the cue direction during the delay period. (d) The difference between the vector direction and the cue direction along the R-ODR trial. The direction of the population vector gradually rotated from the cue direction to the saccade direction during the delay period.
Interactions among prefrontal neurons
Takeda and Funahashi (2006) showed the presence of the information flow from neurons having cue-period activity to neurons having oculomotor activity through neurons having delay-period activity in the DLPFC. While monkeys performed ODR tasks, cue- (C), delay- (D), or response-period (R) activities or their combinations (CD, CR, DR, CDR) were observed in DLPFC neurons. They characterized each neuron based on which task-related activity was exhibited and which information (cue location or saccade direction) each task-related activity represented, then classified these neurons into nine groups (C, Dcue, Dsac, CDcue, DcueRcue, DsacRsac, DcueRsac, CDcueRcue and CDcueRsac). Preferred directions were similar between cue- and delay-period activities in groups of CDcue, CDcueRcue and CDcueRsac, all of which represented visual information, indicating that the directional selectivity of delay-period activity was affected by the directional selectivity of cue-period activity for each neuron. Preferred directions were also similar between delay- and response-period activities in groups of DcueRcue, CDcueRcue and DsacRsac, indicating that the directional selectivity of delay-period activity affects the directional selectivity of response-period activity in these neurons. By the comparison of temporal profiles of delay-period activity among these groups, they found (1) cue-period activity of C, CDcue and CDcueRcue groups might contribute to the initiation and the maintenance of delay-period activity of CDcue, CDcueRcue, Dcue and DcueRcue groups, and (2) saccade-related activity of DsacRsac could be affected by delay-period activity of Dsac and DsacRsac groups. Thus, while monkeys performed the ODR tasks, the information flow from neurons having cue-period activity to neurons having oculomotor activity through neurons having delay-period activity is present in the DLPFC. Information processing could be performed during this information flow process.
Dynamic and flexible changes in functional interaction among temporary information-storage processes would be a key component to understand neuronal mechanisms of information processing. In the DLPFC, Wilson et al. (1994) showed that types of responses (excitatory or inhibitory) of pyramidal and nonpyramidal neurons were often inverted (e.g., pyramidal neurons exhibited excitatory responses while nonpyramidal neurons exhibited inhibitory responses) and that the timing of excitatory and inhibitory responses appear to be phased. This result suggests the presence of functional interactions between pyramidal and nonpyramidal neurons. Rao et al. (1999) used a cross-correlation analysis to examine functional interactions between pyramidal and nonpyramidal neurons in the DLPFC and found inhibitory interactions between pyramidal (p.221) neurons and adjacent nonpyramidal interneurons. Funahashi and Inoue (2000) also applied a cross-correlation analysis to simultaneously isolated pairs of prefrontal activities during ODR performances and examined functional interactions between task-related DLPFC neurons. When both neurons of examined pairs exhibited delay-period activity, these neurons tended to show excitatory interactions and to show similar directional preferences. Interactions between task-related neurons with different directional preferences increased with the progress of the trial. Excitatory interactions among neurons having delay-period activity were frequently observed in the DLPFC, suggesting that the information stored in temporary information-storage processes may be transformed from one form of representation to another or integrated to represent more complex information (e.g., delay-period activity representing a combination of visual cue positions and the temporal order of their presentation) by these interactions.
Each neuron changes the magnitude of activity depending on the trial conditions (e.g., difference in the position where the visual cue was presented or the direction where the saccade was directed), the temporal context of the trial and trial events. Therefore, the strength of functional interactions could be changed depending on the trial conditions or the temporal context of the trial. In fact, the height of the peak of the cross-correlogram changed depending on the cue conditions. This modulation of the peak height in the cross-correlogram was observed in most of the neuron pairs examined in the DLPFC. These results suggest that, although many DLPFC neurons have functional interaction with neurons exhibiting same or different kinds of task-related activity, the strength of the interaction may change dynamically depending on the conditions of the trials. Thus, dynamic and flexible changes of functional interactions among temporary information-storage processes could be an important element in understanding neural mechanisms of information processing.
Two working memory systems
A process-based model of working memory in the DLPFC was explained in the previous section. However, a similar system is also necessary in the brain areas where sensory or motor information are processed. Temporary maintenance of information and processing information could be ubiquitous processes for any kind of information processing in the brain. In fact, tonic sustained delay-period activity has been observed not only in the DLPFC, but also in the frontal eye field (Funahashi et al. 1989; Lawrence et al. 2005; Roesch and Olson 2005), the inferior temporal cortex (Fuster 1990; Fuster and Jervey 1982; Miller et al. 1993; Miyashita and Chang 1988; Naya et al. 1996), the posterior parietal cortex (Chafee and Goldman-Rakic 1998; Murata et al. 1996; Pesaran et al. 2002; Sereno and Amador 2006; Zhang and Barash 2004), and the premotor cortex (Crammond and Kalaska 2000; Kurata and Wise 1988; Obayashi et al. 2003; Weinrich and Wise 1982;). Thus, the process-based model of working memory that we proposed is not just for the model to explain functions of the DLPFC but may be a ubiquitous system in the brain.
The specific feature of working memory system operating in the DLPFC would be that this system is not for information processing of specific purpose, such as only for visual information processing, only for control of limb movements, or only for speech comprehension, but rather is general purpose and is an essential element for a variety of information processing that the DLPFC participates in. The DLPFC is located in the best position to monitor the functional state of the posterior association cortices and to control their functions by sending command signals (or top-down signals), since the DLPFC has heavy anatomical connections, mostly reciprocal connections, with the posterior association cortices. On the other hand, working memory systems must be present in the modality-specific cortical areas, such as the sensory system, as well as the motor system. In these modality-specific cortical areas, temporary maintenance and processing (p.222) of information are also essential processes. Therefore, working memory systems can be divided into two types: a general-purpose working memory system and a modality-specific working memory system.
A modality-specific working memory system is a mechanism for temporary maintenance and processing of certain types of information, such as sensory, linguistic, or motor information. Modality-specific working memory systems will be present in cortical and subcortical areas which participate mostly in sensory and motor information processing. On the other hand, a general-purpose working memory system is a mechanism to maintain or process a variety of information including the behavioral context to perform various cognitive tasks or control signals (e.g., top-down signals) to monitor or regulate operations of other cortical and subcortical areas. The contents of a general-purpose working memory system would affect or regulate the contents of modality-specific working memory systems and lead information processing operated in modality-specific working memory system to necessary directions.
Cohen and others (Cohen and Servan-Schreiber 1992; Cohen et al. 1996; Miller and Cohen 2001) explained prefrontal executive functions by computational architectures with the connectionist framework. They hypothesized that the DLPFC represents the context information, which corresponds to the goal representation in the computational architecture of the production system. Context information can be defined as the information necessary to mediate an appropriate behavioral response, and includes a set of task instructions, a specific prior stimulus, or the result of processing a sequence of prior stimuli (Cohen et al. 1996). To accomplish a particular goal, some brain region needs to maintain an internal representation of the goal, to suppress unnecessary behaviors and to temporally coordinate series of behaviors. Cohen et al. (1996) considered the DLPFC as this cortical region. In this regard, prefrontal executive functions that Cohen and others proposed are similar to the functions of the general-purpose working memory system.
Interactions between the DLPFC and other cortical areas
To understand how the DLPFC acts as the general-purpose working memory system, it is important to consider dynamic and flexible functional interactions between the DLPFC and other cortical and subcortical areas. Corticocortical and corticosubcortical anatomical connections with the DLPFC have been examined extensively – see reviews by Fuster (1997), Petrides (1994), Petrides and Pandya (2002). The DLPFC has reciprocal connections with the posterior parietal cortex, the inferior temporal cortex, the superior temporal polysensory areas, the anterior cingulate, the retro-splenial cortex and the parahippocampal gyrus. The DLPFC also has strong reciprocal connections with the mediodorsal nucleus of the thalamus (Ray and Price 1993; Siwek and Pandya 1991). In addition, the DLPFC has connections with the frontal eye field, the presupplementary motor area, the premotor cortex, and the caudate nucleus (Miyachi et al. 2005). Morris et al. (1999) reported that the mid-dorsolateral prefrontal cortex (areas 46 and 9) projects to the retrosplenial area 30 and the posterior presubiculum, and suggested that this system could be the anatomical substrate of functional interaction between the DLPFC and the hippocampus. Thus, the DLPFC has anatomical connections with various cortical and subcortical areas, especially sensory and motor association areas and the limbic brain areas. Most of these connections are reciprocal. These observations indicate that the DLPFC can receive various kinds of information including sensory, motor, and emotional information as well as information stored in long-term memory, and is located in a good position to monitor both internal and external environment. These observations also indicate that the DLPFC can send various kinds of information and signals to various brain areas, and is again located in a good position to coordinate information processing operated in various brain regions and direct these operations to a particular goal. Strong reciprocal (p.223) connections between the DLPFC and various brain areas are the anatomical basis of dynamic and flexible interactions between the general-purpose working memory system and the modality-specific working memory systems.
Noninvasive brain imaging studies have also revealed dynamic and flexible functional interactions between the DLPFC and other cortical areas. n-back tasks have often been used as working memory tasks. Bilateral DLPFC has been shown to be activated in visuospatial n-back tasks (Cohen et al. 1997; Smith and Jonides 1997, 1998). The magnitude of the activation in the DLPFC depended on the memory load required to perform the task (Callicott et al. 1999; Carlson et al. 1998). However, working memory tasks like n-back tasks are composed of numerous elementary neural processes. Based on the analysis using evoked potentials, McEvoy et al. (1998) showed that, although some of these neural processes were affected by the type of information (verbal or spatial), the neural processes associated with frontal and parietal slow waves were affected not by the type of information, but by the amount of information being retained. McEvoy et al. (1998) suggested that working memory is operating by dynamic cortical networks of information-specific processes (e.g., modality-specific working memory system) and nonspecific, capacity-limited, higher-order processes (e.g., general-purpose working memory system).
Dynamic cortical networks including the DLPFC have been shown directly by correlation analyses in functional brain imaging studies as well as by covariance and correlation analyses of event-related potentials. Using a working memory task of faces, McIntosh et al. (1996) examined functional interactions among different cortical regions by an anatomy-based covariance structural equation model. As a result, they found that different functional interactions among different cortical areas were observed in different task conditions (e.g., different delay lengths) and that a top-down interaction from the DLPFC to the temporal and occipital cortices was observed in a long delay condition. McIntosh et al. (1999) also showed that only aware subjects exhibited learning-related modulations of brain activation in the left DLPFC during an associative learning task and that a correlation analysis of this modulation revealed significant functional connectivity among bilateral DLPFC, the sensory association cortices and the cerebellum. In addition, evidence for homotopic interaction or coactivation of the bilateral DLPFC has been reported (Klingberg et al. 1997; McGuire et al. 1991). Klingberg et al. (1997) reported the increase of bilateral coactivation of the DLPFC and the inferior parietal cortex in association with the increase of task difficulty. They also found that the inferior parietal cortex was often coactivated with the DLPFC in working memory tasks and tasks involving planning. Further, Huettel et al. (2005) showed that the degree of uncertainty on decision making influenced the magnitude of activation of both prefrontal and parietal cortices. Collette et al. (2005) showed activation of both the prefrontal and the inferior parietal cortex in dual-task performance. Thus, all of these results indicate the importance of functional interactions as well as coactivation between the DLPFC and other cortical and subcortical areas when subjects perform cognitive tasks. These results also indicate that functional interactions and coactivation between the DLPFC and other cortical and subcortical areas are dynamically and flexibly modulated depending on the context of the cognitive task.
Evidence of top-down modulatory effects by the DLPFC have also been shown. Using visual associative memory tasks, Hasegawa et al. (1998) showed that retrieval of visual images from long-term memory was under the executive control of the DLPFC by an experiment using partial split-brain monkeys. In addition, by neurophysiological experiments using monkeys having resection of the corpus callosum, Tomita et al. (1999) showed that top-down signals from the DLPFC regulated retrieval of associative memory in inferior temporal neurons. These observations indicate that the DLPFC can control information processing in other cortical areas by sending top-down signals (Miyashita 2005). Lumer and Rees (1999) showed by fMRI that covariation of activation was observed in multiple extrastriate areas, the parietal cortex, and the prefrontal (p.224) cortex in bistable viewing conditions. Coordinated activation of these areas was not linked to either sensory or motor events, but rather reflected perceptual events or internal changes of perception. Therefore, they concluded that functional interactions between visual areas and the prefrontal cortex are important for conscious vision. On the other hand, either enhancement or suppression of the visual response was observed in inferior temporal neurons when two visual stimuli (one preferred and the other nonpreferred) were presented simultaneously in the neuron’s receptive field. Chelazzi et al. (1998) proposed that this phenomenon can be explained by a ‘biased competition’ model of attention. Based on this model, simultaneously presented objects in the visual receptive field would compete with others for representation within the cortex. They suggested that this competition could be biased by ‘top-down’ feedback signals from brain structures involved in working memory. Based on the results by Hasegawa et al. (1998), Tomita et al. (1999), and Lumer and Rees (1999), it would be reasonable to think that top-down signals from the DLPFC bias the magnitude of the activity in the visual system. These results indicate the presence and importance of top-down modulatory effects by the DLPFC to perform cognitive tasks. These results also suggest that top-down modulatory effects by the DLPFC affect a wide variety of cognitive activities including sensory perception, directed attention, episodic memory encoding and retrieval through dynamic and flexible functional interactions. This could be the typical function of the DLPFC as the general-purpose working memory system.
Conclusions
In this manuscript, we proposed a general-purpose working memory system to explain how the DLPFC performs executive control. This general-purpose working memory system can (1) represent and process various kinds of information, (2) access various systems (e.g., modality-specific working memory systems) to obtain any kind of necessary information and monitor their operations, and (3) send appropriate information and signals (e.g., top-down signals) to various systems to control their operations. To represent and process various kinds of information in the general-purpose working memory system, this system needs to integrate following four processes: a selection process, a temporary information-storage process, an output process and modulatory signals. We also proposed the presence of extensive functional interactions among temporary information-storage processes for information processing. The dynamic and flexible nature of these interactions is an essential feature of the general-purpose working memory system. In addition, dynamic and flexible interactions between the general-purpose working memory system and the modality-specific working memory systems are also essential to consider the function of the general-purpose working memory system as well as the functions of the DLPFC. However, it is not known how the selection process selects necessary information from a variety of information in a variety of brain areas, how the DLPFC creates appropriate top-down signals, how the DLPFC sends appropriate information and top-down signals to the exact systems or exact brain areas to control their operations, and how the DLPFC can manage a variety of functions efficiently. Therefore, we need further experiments to solve these questions and to understand functions of the DLPFC as the general-purpose working memory system.
Acknowledgment
This work was supported by a Grant-in-Aid for Scientific Research (No. 17021022, 17300103, 17650101) from the Japanese Ministry of Education, Culture, Sports, Science, and Technology (MEXT). This work was also supported by the 21st Century COE Program (D-2 to Kyoto University) by the MEXT.
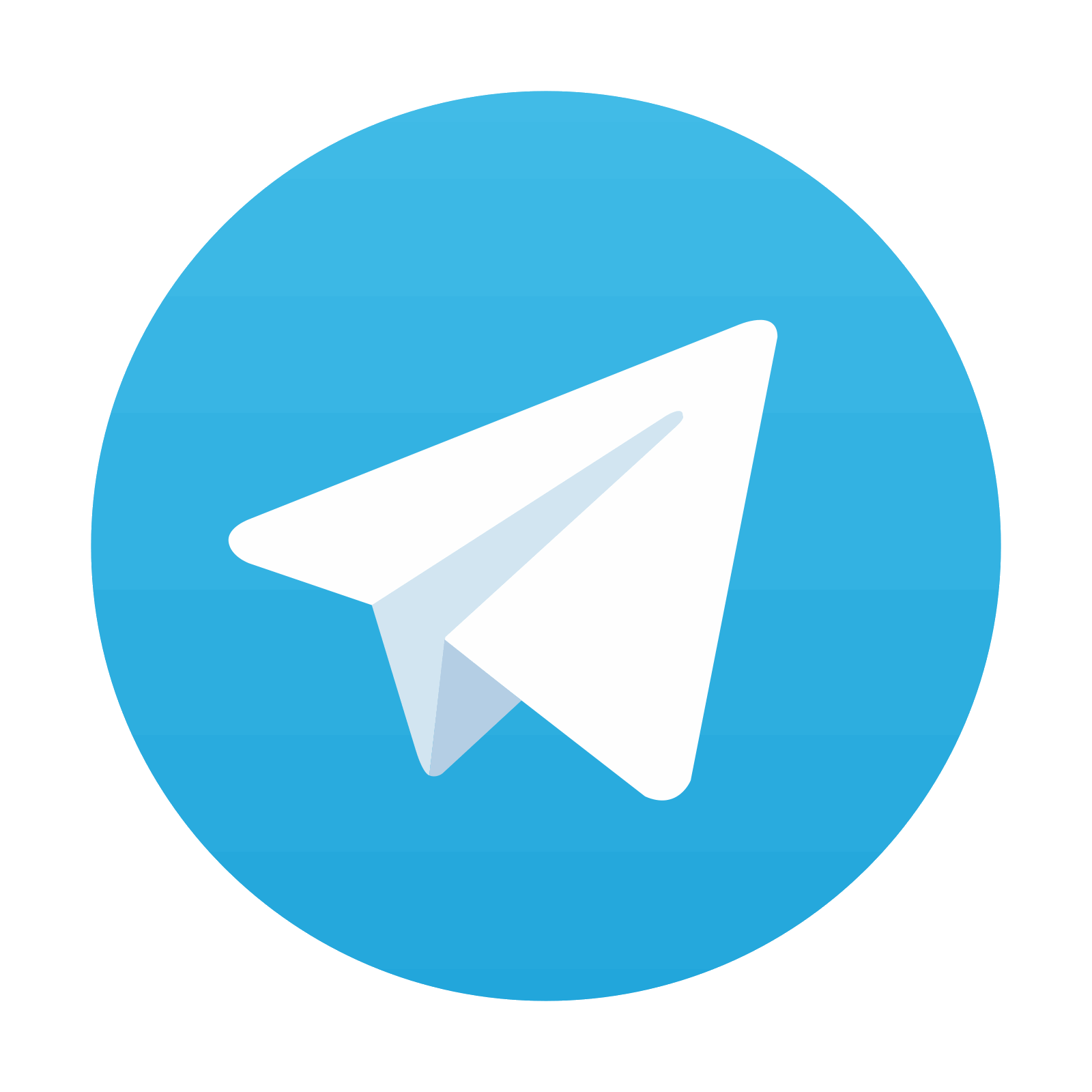
Stay updated, free articles. Join our Telegram channel
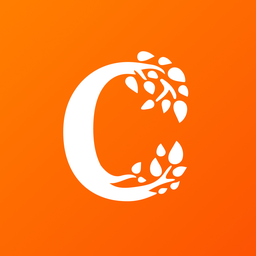
Full access? Get Clinical Tree
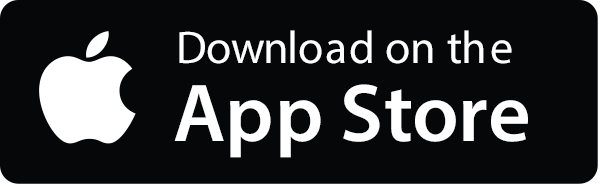
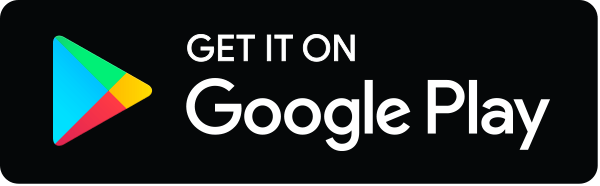