CLINICAL CASE | Lateral Medullary Syndrome and Horner Syndrome
A 69-year-old hypertensive man suddenly developed vertigo and left facial numbness. He is unable to stand unassisted.
His sensory and motor functions were tested at the emergency room. Pain and temperature sensations are markedly decreased on the left side of his face, including the left side of his oral cavity. Tactile sensation is preserved bilaterally on his face. Pain and temperature sensations are diminished on the right side of the scalp, neck, limbs, and trunk. Touch and limb propriosensation were normal bilaterally. There is a loss of the gag reflex on the left.
On finger-nose-finger testing of the left arm and heel-to-shin testing of the left leg, his movements are ataxic. He has difficulty making rapidly alternating movements (dysdiadochokinesia) with the left arm. Corresponding right limb functions are normal. He has difficulty standing, and the limited walking he is able to accomplish is associated with a broad-based gait. His voice sounds hoarse. He is able to extend his tongue along the midline.
On further examination, the patient also is noted to have mild ptosis on the left. His pupils were reactive to light, but his left pupil was smaller than the right. Finally, the left side of his face feels dry and warm to touch.
Figure 15–1A is an MRI of the medulla, and Figure 15–1B, a nearby myelin-stained section. The bright dorsolateral region in part A is the site of an infarction.
Answer the following questions on the basis of your reading of this chapter and prior chapters on sensory and motor functions of the dorsolateral medulla.
1. Occlusion of which artery would infarct the medullary region shown on the MRI?
2. Indicate the particular nucleus or tract that, after damage by infarction, produces: (1) ipsilateral facial pain loss, (2) contralateral loss of limb and trunk pain, (3) ataxia, (4) hoarse voice, and (5) ipsilateral ptosis.
Key neurological signs and corresponding damaged brain structures Distribution of the posterior inferior cerebellar arteryThe site of lesion corresponds to the distribution of the posterior inferior cerebellar artery. The territory supplied by this artery receives little collateral circulation (see Chapter 3). This means that blood flow from a functioning neighboring artery does not take over, as in many regions of the brain. Remaining areas of the medulla at this level are supplied by small, direct branches from the vertebral artery.
Alternating loss of pain and temperature on the left side of the face and right limbs and trunk with preservation of touchThe lesion produced a classical sign. Ipsilateral loss of facial pain and temperature sensation is due to interruption of the spinal trigeminal tract, as well as part of the spinal trigeminal nucleus (caudal nucleus). Contralateral loss of pain and temperature sensation on the neck, limbs, and trunk is due to interruption of the anterolateral system, which decussates in the spinal cord (Figure 15–1C). This pattern was considered in the Chapter 6 clinical case in which tactile and limb position senses are preserved because the dorsal column-medial lemniscal system is located outside of the distribution of the posterior inferior cerebellar artery.
Hoarse voiceSeveral cranial nerve motor nuclei are located within the territory of the posterior inferior cerebellar artery; especially nucleus ambiguus, which contains pharyngeal and laryngeal motor neurons. The hoarse voice is produced by unilateral paralysis of laryngeal muscles. The patient is unable to close off the trachea and build pressure within the lungs, for his voice to project. This lesion often disrupts the laryngeal closure reflex, in which the vocal cords close when the surrounding region is stimulated by solid food or liquid. Normally, this reflex prevents aspiration of materials into the lungs (see Chapter 11). The loss of the gag reflex is likely due to lesion of the nucleus ambiguus, although the solitary nucleus and trigeminal nuclei are affected, which can impede the function of the afferent limb of the reflex, which is carried by the glossopharyngeal nerve. There may also be swallowing impairments, but this was not tested.
Ataxia and dysdiadochokinesiaThree major inputs to the cerebellum travel through the inferior cerebellar peduncle, which is supplied by the posterior inferior cerebellar artery: climbing fibers from the inferior olivary nucleus and the cuneocerebellar and dorsal spinocerebellar tracts. The cuneocerebellar tract originates from within the infarcted zone. Loss of these key cerebellar paths leads to the observed motor signs. Moreover, the posterior inferior cerebellar artery also supplies parts of the cerebellar cortex and deep nuclei. Thus, these signs can be due to direct involvement of the cerebellum, in addition to its input pathways. Strength is usually preserved after infarction of the posterior inferior cerebellar artery. This is because the corticospinal tract travels in the pyramids, which are not supplied by the posterior inferior cerebellar artery.
VertigoVertigo is a classic sign of vestibular nerve and nuclei damage. This results because of an imbalance in vestibular signaling. However, cerebellar involvement can produce vertigo. Damage to the dorsolateral medulla and cerebellum can often produce nystagmus.
Pupillary signs, ptosis, and facial dryness and warmthPupils and eyelid control are functions of the midbrain. How can they be affected with a medullary lesion? This is because the descending pathway from the hypothalamus to the spinal cord that controls aspects of sympathetic nervous system function travels in the dorsolateral medulla (see Figure 15–1B; dashed circle). The sympathetic nervous system produces pupillary dilatation. Pupillary constriction occurs in the patient because of the loss of sympathetic drive to the ciliary muscle, and now, unopposed actions of the parasympathetic nervous system. Mild ptosis (termed pseudoptosis) is due to the loss of sympathetic control of smooth muscle that assists the mechanical action of the levator palpebrae muscle. Sweating is also a sympathetic nervous system function; its loss produces dryness. Loss of sympathetic vasoconstriction results in arterial vasodilation.
ReferenceBrust JCM. The Practice of Neural Science. New York, NY: McGraw-Hill; 2000.
Choi K-D, Oh S-Y, Park S-H, Kim J-H, Koo J-W, Kim JS. Head-shaking nystagmus in lateral medullary infarction. Neurology. 2007;68:1337-1344.
Kim JS, Moon SY, Park S-H. Ocular lateropulsion in Wallenberg syndrome. Neurology. 2004;62:2287.
The hypothalamus is crucial for maintaining normal organ function and for producing many of the behaviors necessary to meet basic needs such as feeding, drinking, mating, and sleeping. The hypothalamus thus ensures survival of both the individual and its species. Virtually every body organ depends on the hypothalamus for some aspect of control, including the heart, lungs, gastrointestinal tract, and genitourinary organs. The hypothalamus helps organize the body’s reactions to disease, such as fever production in response to infection. Skeletal muscle depends on the hypothalamus for regulating its blood supply; even bone mass seems to depend on hypothalamic regulation. In animals, the hypothalamus controls complex behaviors, like sexual responsiveness, maternal care (eg, nursing), and aggression. Whereas it can only be speculated how much of similar human behaviors depend on the hypothalamus, it is now becoming clear that the hypothalamus is important in regulating aspects of human social behavior. The list goes on and on! Compared with just about every other region of the nervous system, each of which serves a small set of functions, the hypothalamus accomplishes a bewilderingly wide range of tasks. We will see that it does this largely by using information about the body’s internal state, about emotions, and about critical environmental stimuli, to control hormone production and the autonomic nervous system, and to regulate the moment-to-moment functions of neural circuits for arousal.
This chapter first considers hypothalamic gross anatomy. Next, the chapter surveys its functional organization, highlighting several key aspects. Additional important functions of the hypothalamus are examined when we learn about its regional anatomy, later in the chapter. Chapter 16 revisits the hypothalamus and its role in motivational and appetitive behavior, along with other brain structures that comprise the limbic system for emotions.
The hypothalamus is a tiny diencephalic structure, about 1-cm square, that is located ventral and anterior to the thalamus (Figure 15–2). The third ventricle separates the two halves of the hypothalamus. On its medial, or ventricular, surface the hypothalamus is distinguished from the thalamus by a shallow groove, the hypothalamic sulcus. Anteriorly, part of the hypothalamus extends a bit beyond the anterior wall of the third ventricle. (The anterior wall of the third ventricle is the location of the most anterior portion of the developing central nervous system, or the lamina terminalis; Figure 15–2.) The hypothalamus reaches caudally, just beyond the mammillary bodies, paired hypothalamic nuclei on its ventral surface (Figure 15–3).
We will learn that the functions of the hypothalamus are organized by projection neurons in discrete nuclei, or small groups of nuclei, that interface with different effector systems and circuits in other parts of the central nervous system. These hypothalamic nuclei are arranged into three mediolateral zones, each with distinctive functions (Figures 15–3 and 15–4; Table 15–1):
FIGURE 15–4
A. The major nuclei are illustrated in a cutaway view of the hypothalamus. The inset shows the region illustrated in the cross-sectional view (B). The arcuate and periventricular nuclei comprise the periventricular zone; they form a thin veil beneath the walls and floor of the third ventricle. The middle and lateral zones are the two other hypothalamic zones. The line shows the approximate plane of section in B. B. Myelin-stained coronal section through the hypothalamus. The approximate locations of the key hypothalamic nuclei are shown. The periventricular and arcuate nuclei comprise the periventricular zone. The other nuclei form the middle and lateral hypothalamic zones.

Nucleus | Mediolateral zone | Key functions |
---|---|---|
Anterior Hypothalamus | ||
Preoptic nuclei: | ||
Ventrolateral | Lateral | Sleep-wakefulness |
Medial | Periventricular | Parvocellular hormone control |
Middle | Thermoregulation | |
Middle Hypothalamus | ||
Paraventricular | Periventricular and middle | Magnocellular hormones (oxytocin, vasopressin); parvocellular; direct autonomic control, including urination |
Supraoptic | Middle | Magnocellular hormones (oxytocin, vasopressin) |
Arcuate | Periventricular | Parvocellular hormones; visceral functions |
Suprachiasmatic | Middle | Circadian rhythm |
Ventromedial | Middle | Appetitive/consummatory behaviors |
Dorsomedial | Middle | Feeding, drinking, and body weight regulation |
Periventricular | Periventricular | Parvocellular hormones |
Posterior Hypothalamus | ||
Mammillarya | Memory | |
Tuberomammillary | Lateral | Sleep-wakefulness (histamine) |
Lateral Hypothalamusb | ||
Lateral hypothalamic and perifornical areas | Lateral | Various, including arousal, food intake; contain orexin |
The periventricular zone is the most medial and comprises thin nuclei that border the third ventricle. This zone is important in regulating the release of endocrine hormones from the anterior pituitary gland.
The middle zone, which is interposed between the periventricular and lateral zones, serves diverse functions. It contains nuclei that regulate the release of vasopressin and oxytocin from the posterior pituitary gland. It is also a major site for neurons that regulate the autonomic nervous system. The body’s biological clock is set by neurons in this zone, as are aspects of the control of wakefulness.
The lateral zone is separated from the medial zone by the fornix, a C-shaped tract that interconnects limbic system structures (see Figure 1–11A). This zone contains neurons that integrate information from other hypothalamic nuclei and telencephalic structures engaged in emotions. This is also a region important for regulating sleep and wakefulness and feeding.
The nuclei of the hypothalamus are located at discrete anterior-posterior positions (Table 15–1). This describes an orthogonal regional organization that will be the basis for examining sections through the hypothalamus later in the chapter. Historically, this organization was stressed because scientists recognized distinctive differences between the functions of the anterior and posterior hypothalamus. Now, we recognize these differences in the context of discrete hypothalamic nuclei rather than its gross anatomy. Whereas the boundaries between the anterior-posterior regions are not precise, a general knowledge of their locations is essential for an understanding of the three-dimensional organization of the hypothalamus.
The anterior part of the hypothalamus, located dorsal and rostral to the optic chiasm (see Figure 15–3, inset), includes the preoptic area, with its numerous preoptic nuclei. The preoptic area is sometimes considered separate from the hypothalamus. But because its functions are intimately related to other, more caudal, hypothalamic functions, it is best to consider them together. The principal nucleus for circadian rhythms is also located in the anterior hypothalamus.
The middle hypothalamus is between the optic chiasm and the mammillary bodies. This portion contains the infundibular stalk, from which the pituitary gland arises. The nuclei for anterior and posterior pituitary hormone release are mostly located here.
The posterior portion includes the mammillary bodies and nuclei dorsal to them.
Separate Parvocellular and Magnocellular Neurosecretory Systems Regulate Hormone Release From the Anterior and Posterior Lobes of the Pituitary
The pituitary gland is connected to the ventral surface of the hypothalamus by the infundibular stalk (Figure 15–4A). In humans, two major anatomical divisions of the pituitary gland mediate the release of distinct sets of hormones (Figure 15–5): the anterior lobe (also called the adenohypophysis; see Table 15–2) and the posterior lobe (or neurohypophysis). A third lobe, the intermediate lobe, although prominent in many simpler mammals, is vestigial in humans.
Anterior pituitary hormones | Releasing hormones (RH) | Release-inhibiting hormones (RIH) |
---|---|---|
Growth hormone | Growth hormone RH | Somatostatin (growth hormone RIH) |
Lutenizing hormone | Gonadotrophin RH | |
Follicle-stimulating hormone | Gonadotrophin RH | |
Thryotropin | Thryotropin RH (or TRH) | Somatostatin (growth hormone RIH) |
Prolactin | Prolactin RH | Prolactin RIH; dopamine |
Adrenocorticotropic hormone (ACTH) | Corticotropin RH (CRH) | |
Melanocyte-stimulating hormone (MSH) | Melanocyte-stimulating hormone RH | Melanocyte-stimulating hormone RIH |
The anterior and posterior lobes are parts of two distinct neurosecretory systems, and hormone release from these lobes is regulated by different populations of hypothalamic neurons. The anterior lobe is part of the parvocellular neurosecretory system (Figure 15–5A). This system contains small-diameter hypothalamic neurons (hence the term parvocellular) that are located in numerous nuclei. They regulate hormone release by epithelial secretory cells of the anterior pituitary. Parvocellular neurosecretory neurons are located predominantly in nuclei of the periventricular zone. By contrast, the posterior lobe is part of the magnocellular neurosecretory system (Figure 15–5B). Here, axons of large-diameter hypothalamic neurons in two nuclei project to and release peptide hormones into the posterior lobe. Rostrocaudally, parvocellular neurosecretory neurons are located in all the three hypothalamic regions, whereas the magnocellular neurosecretory neurons are mostly located in the middle zone.
Regulatory peptides released into the portal circulation by hypothalamic neurons control secretion of anterior lobe hormones
The process by which the hypothalamus stimulates anterior lobe secretory cells to release their hormones (or to inhibit release) is quite unlike mechanisms of neural action considered in earlier chapters. Rather than synapse on anterior lobe secretory cells, the hypothalamic parvocellular neurosecretory neurons terminate on capillaries of the pituitary portal circulation in the floor of the third ventricle (Figure 15–5A).
A portal circulatory system is distinguished by the presence of separate portal veins interposed between two sets of capillaries. The first set is located in a region termed the median eminence, which is part of the proximal infundibular stalk. The portal veins are located in the distal part of the infundibular stalk. The second set of capillaries is found in the anterior pituitary. (In the systemic circulation, such as the vascular supply of the rest of the brain, capillary beds are interposed between arterial and venous systems.)
Parvocellular neurons release chemicals, most of which are peptides, that either promote (releasing hormones) or inhibit (release-inhibiting hormones) the release of hormones from anterior lobe secretory cells (Table 15–2). Release or release-inhibiting hormones are carried to the anterior lobe in the portal veins (Figure 15–5A), where they act directly on epithelial secretory cells.
An analogy can be drawn between the capillaries in the median eminence and the integrative function of spinal motor neurons (see Chapter 10). Separate descending pathways and spinal interneuronal systems synapse on the motor neuron. Thus, the motor neuron is the final common pathway for the integration of neuronal information controlling skeletal muscle. The final common pathway for control of anterior lobe hormone release comprises the capillaries of the median eminence. This is because different hypothalamic neurons secrete releasing or release-inhibiting hormones into the capillaries of the median eminence (Figure 15–5A), and summation of neurohormones occurs at this vascular site.
The distribution of neurons that project to the median eminence has been examined extensively in rodents. Although these neurons are widespread, the major sources are located in nuclei within the periventricular zone (Figures 15–4 and 15–5A). Among the major sources, and some of the hormones they release, are the following:
The arcuate nucleus contains neurons that release gonadotropin-releasing hormone, luteinizing hormone–releasing hormone, somatostatin, and adrenocorticotropic hormone.
Neurons in the periventricular portion of the parvocellular nucleus (the part that lies along the third ventricle) contain corticotropin-releasing hormone (CRH).
The periventricular nucleus provides gonadotropin-releasing hormone, luteinizing hormone–releasing hormone, and dopamine (which inhibits prolactin release).
The medial preoptic area contains parvocellular neurons that secrete luteinizing hormone–releasing hormone.
In addition, there are extrahypothalamic sources of releasing and release-inhibiting neurohormones. For example, the septal nuclei (see Chapter 16) are a source of gonadotropin-releasing hormone. Interestingly, many of these neurohormones are also found in hypothalamic neurons that do not project to the median eminence and in neurons in other regions of the central nervous system. This widespread distribution of neurohormones indicates that they are neuroactive compounds at these other sites and not just chemicals that regulate anterior pituitary hormone release. Individual neurons of the parvocellular system, as in the magnocellular system (see below), may synthesize and release more than one peptide. This peptide synthesis and release may be regulated by circulating hormones in the blood. This is one way in which environmental factors, such as prolonged exposure to stressful situations, may alter the neurohormonal composition in the portal circulation and thereby influence anterior pituitary hormone release. Note that the blood-brain barrier is less of an obstacle in the hypothalamus than in most other brain regions (see Figure 3–16B).
Posterior pituitary hormones, vasopressin and oxytocin, are the neurosecretory products of hypothalamic neurons; they have diverse functions on body organs. The peptide vasopressin elevates blood pressure, for example, through its action on vascular smooth muscle. Vasopressin also promotes water reabsorption from the distal tubules of the kidney to reduce urine volume. Another name for vasopressin is antidiuretic hormone (sometimes called ADH). Oxytocin is a peptide with a chemical structure nearly identical to that of vasopressin, differing by amino acids at only two sites. Oxytocin is best known for its actions on female organs, where it functions to stimulate uterine contractions and to promote ejection of milk from the mammary glands. There are other important behavioral actions of vasopressin and oxytocin. Both are important for pair bonding in monogamous animals of both sexes, although most studies focus on oxytocin in females and vasopressin in males. Both peptides also are important in other aspects of social behavior, such as vasopressin in social recognition and oxytocin in formation of interpersonal trust.
In the hypothalamus, both vasopressin and oxytocin are synthesized primarily in two nuclei, the paraventricular nucleus and the supraoptic nucleus (Figure 15–5B). Experiments in animals have shown that the paraventricular nucleus comprises at least three distinct cell groups. As described earlier, there are parvocellular neurosecretory neurons in the portion of the nucleus that apposes the third ventricle. Lateral to these neurons are the magnocellular neurosecretory neurons that synthesize and release the two posterior lobe neurohormones. A third neuron group, typically considered magnocellular because of their morphology not hormonal function, gives rise to a descending brain stem and spinal projection for regulating autonomic nervous system functions (see next section). The supraoptic nucleus consists only of magnocellular neurosecretory neurons. However, a small number of oxytocin-containing neurons of both the paraventricular and supraoptic nuclei project to several other brain regions, where they are thought to regulate aspects of social behavior.
Both vasopressin and oxytocin are synthesized from larger prohormone molecules. The prohormone molecules from which vasopressin and oxytocin derive contain additional proteins, called neurophysins. It was once thought that vasopressin was synthesized in one nucleus and oxytocin in the other. With the use of immunocytochemical techniques, however, it has been established that different cells in each nucleus produce one or the other hormone.
The axons of the paraventricular and the supraoptic magnocellular neurons in the infundibular stalk (Figures 15–4A and 15–5B) do not make synaptic contacts with other neurons. Rather, they terminate on fenestrated capillaries in the posterior lobe of the pituitary. (Fenestrations, or pores, make capillaries leaky. Recall that the posterior lobe of the pituitary [see also Figure 3–16] is one of the brain regions lacking a blood-brain barrier. Thus, neurohormones can pass freely into the capillaries through the fenestrations.)
Immunocytochemical studies also have shown that magnocellular neurons, like their parvocellular counterparts, contain other peptides that act on neurons in the central nervous system and on peripheral organs. These other peptides also may be released into the circulation along with oxytocin or vasopressin and have coordinated actions on diverse structures. Vasopressin itself is an example of a brain peptide that has a diversity of coordinated functions at different sites. For example, it is a blood-borne hormone that influences the function of specific peripheral target organs, such as the kidney, and it is a neuroactive peptide involved in control of the autonomic nervous system (see below).
An understanding of the projections from other brain regions to magnocellular hypothalamic neurons provides insight into how the brain controls neurohormone release. For example, magnocellular neurons that contain vasopressin are important for regulating blood volume. These neurons receive inputs from three key sources that each serve a related function.
First, magnocellular neurons receive an indirect projection from the solitary nucleus. This pathway conveys baroreceptor input from the glossopharyngeal and vagus nerves (see Chapter 6) to the hypothalamus, providing important afferent signals for controlling blood pressure and blood volume.
The second major input source is from two circumventricular organs, the subfornical organ and the organum vasculosum of the lamina terminalis (see Figure 3–16). The circumventricular organs do not have a blood-brain barrier. As discussed in Chapter 3, the blood-brain barrier is a specific permeability barrier between capillaries in the central nervous system and the extracellular space. This barrier protects the brain from the influence of many neuroactive chemicals circulating in the blood. Without a blood-brain barrier, neurons in subfornical organ and the organum vasculosum of the lamina terminalis are capable of sensing plasma osmolality and circulating chemicals and thereby can regulate blood pressure and blood volume through their hypothalamic projections.
The preoptic area provides the third input to the magnocellular neurons. This region is implicated in the central neural mechanisms for regulating the composition and volume of body fluids and thus indirectly affects the control of blood pressure.
The Parasympathetic and Sympathetic Divisions of the Autonomic Nervous System Originate From Different Central Nervous System Locations
The hypothalamus regulates the autonomic nervous system. The autonomic nervous system controls several organ systems of the body: cardiovascular and respiratory, gastrointestinal, exocrine, and urogenital. Two divisions of the autonomic nervous system—the parasympathetic and sympathetic nervous systems—originate from different parts of the central nervous system. Similar to the control of skeletal muscle, visceral control by the sympathetic and parasympathetic systems relies on both relatively simple reflexes, involving the spinal cord and brain stem, and more complex control by higher levels of the central nervous system, especially the hypothalamus.
The enteric nervous system is sometimes considered a third division of the autonomic nervous system. It is located entirely in the periphery. This system provides the intrinsic innervation of the gastrointestinal tract and mediates the complex coordinated reflexes for peristalsis. It is thought that the enteric nervous system functions independent of the hypothalamus and the rest of the central nervous system.
The next section reviews the anatomical organization of the sympathetic and parasympathetic divisions. An understanding of how these autonomic divisions connect to their target organs is essential before considering their higher-order regulation by the hypothalamus.
Sympathetic and parasympathetic system innervation of body organs differs from the way the somatic nervous system innervates skeletal muscle
The innervation of skeletal muscle is mediated directly by motor neurons located in spinal and cranial nerve motor nuclei (Figure 15–6, left side of spinal cord). Further, skeletal muscle is controlled primarily by the contralateral cerebral cortex (Figure 15–6, red line) and various brain stem motor control nuclei (see Chapter 10). For the autonomic innervation of the viscera, two neurons link the central nervous system with organs in the periphery: the preganglionic neuron and the postganglionic neuron. Visceral control is mediated by the ipsilateral hypothalamus (Figure 15–6, black line) and brain stem nuclei. This is shown for the sympathetic nervous system in Figure 15–6 (right side of spinal cord; see Figure 11–4); more is known about the central control of the sympathetic than parasympathetic nervous system. The cell body of the sympathetic preganglionic neuron is located in the central nervous system, and its axon follows a tortuous course to the periphery. From the ventral root and through various peripheral neural conduits, the axon of the preganglionic neuron finally synapses on postganglionic neurons in peripheral ganglia (Figure 15–6). A notable exception is the adrenal medulla, which receives direct innervation by preganglionic sympathetic neurons. This exception is related to the fact that adrenal medullary cells, like postganglionic neurons, develop from the neural crest (see Chapter 6).
FIGURE 15–6
The circuits for skeletal muscle control and visceral organ innervation by the sympathetic nervous system. For the viscera, control is exerted primarily from the hypothalamus. Preganglionic sympathetic autonomic neurons are located in the intermediate zone of the spinal cord. Their axons exit the spinal cord through the ventral roots and project to ganglia in the sympathetic trunk (paravertebral ganglia) through the spinal nerves and white rami. The axons of postganglionic neurons in the sympathetic ganglia course to the periphery through the rami and spinal nerves. The white and gray rami contain, respectively, the myelinated and unmyelinated axons of preganglionic and postganglionic autonomic neurons. A postganglionic neuron in a prevertebral ganglion is also shown with input from a preganglionic neuron. For somatic muscle control, control derives from the descending motor paths; the corticospinal tract is shown. Muscle is innervated directly by motor neurons in the ventral horn. Note that for skeletal muscle control, only the direct path from the cerebral cortex to motor neurons is shown. In addition, there are indirect paths that relay in the brain stem and via spinal cord interneurons.
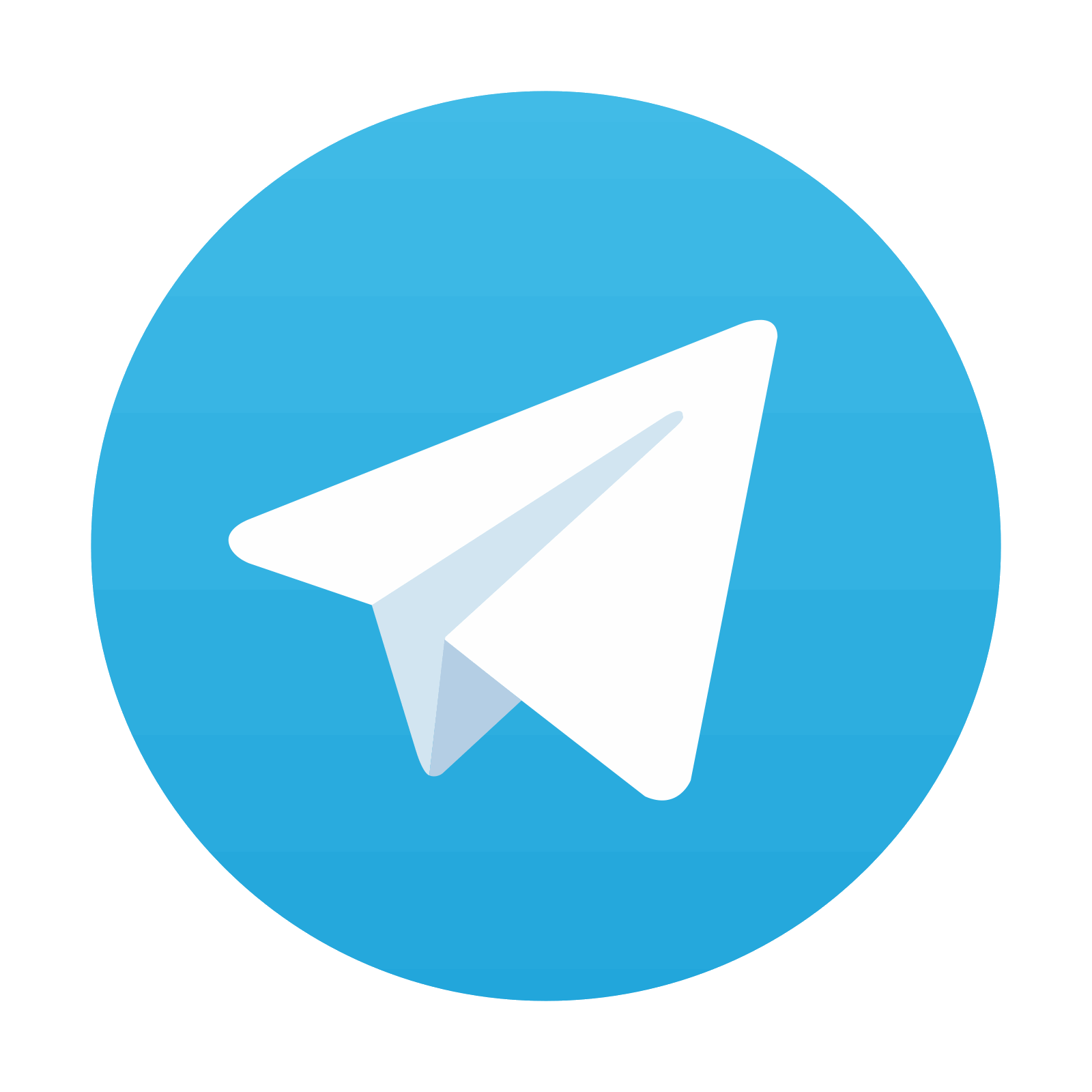
Stay updated, free articles. Join our Telegram channel
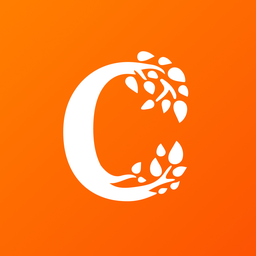
Full access? Get Clinical Tree
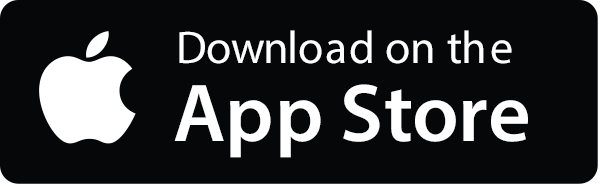
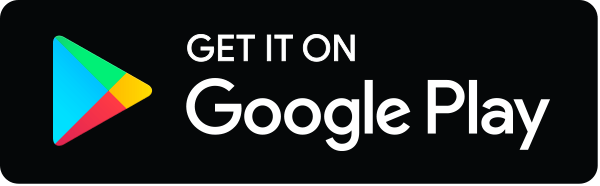
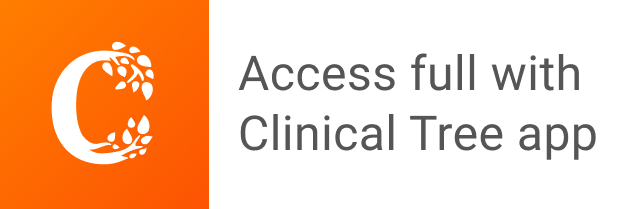