Fig. 5.1
A dendrogram depicting phylogenetic relationships for nine species from the mammalian clade Boreoeutheria. Minicolumn widths were derived from the frontal lobe using the pyramidal cell array as a defining feature (The following sources were used in collating data for Figs. 5.1, 5.2, and 5.3: Escobar et al. 1986; White and Peters 1993; Peters and Yilmaz 1993; Feldman and Peters 1974; Favorov and Diamond 1990; Tommerdahl et al. 1993; Gabbott and Bacon 1996; Peters and Walsh 1972; Peters and Kara 1987; Kohn et al. 1997; Fleischauer et al. 1972; DeFelipe et al. 1990; Peters and Sethares 1991, 1996, 1997; Buxhoeveden et al. 2001a; Von Bonin and Mehler 1971; Kaas et al. 1981; Buxhoeveden and Casanova 2002a; Schlaug et al. 1995; Seldon 1981; del Rio and DeFelipe 1997a, b; Buldyrev et al. 2000; and Casanova and Tilquist 2008. Note that in certain cases an average minicolumn width had been computed by calculating the mean based on the minimum and maximum values reported in each study)
The comparative approach also reveals homoplasies, suggesting the independent emergence of a character or character state that is unrelated to ancestry but rather a result of similar environmental or ecological pressures. For example, in the comparison shown in Fig. 5.1, humans, domestic cats, and rats share a convergent pattern of enlarged minicolumn width that likely arose independently in these species. It is also important to point out in this broad comparative framework, that overall neocortical size does not appear to be typically associated with minicolumn widths across the range of mammalian phylogeny. The comparative approach thus challenges us to explore the various ways in which the cortical column differs among species and how these differences are manifest functionally.
A framework that embraces diversity in minicolumn structure would also enable the recognition of certain general organizational properties and identify constraints that limit evolutionary change in the cortical column. When combined with ecological, life history or brain: body size data, this can also be a powerful tool for exploring relationships between minicolumn structure and other aspects of species’ biology. For instance, minicolumns have not been investigated across a wide range of brain sizes. Large comparative studies could establish the limits for cortical column dimensions and reveal if there exist patterns related to gyrencephaly or brain size (Buxhoeveden and Casanova 2002a, b). Figure 5.2 shows the scaling of minicolumn width (using the pyramidal cell array) to brain mass as reported in the literature for a range of anthropoid primate species encompassing ~30 Ma of evolutionary diversification. As indicated by this preliminary analysis, minicolumn width in anthropoid primates has a positive and significant allometry with brain size, i.e., with every gram increase in brain size, minicolumn width increases by 0.012 μm, suggesting that while slight, brain mass is a significant determinant of overall minicolumn size within this phylogenetic lineage and, as a result, a determinant of variation in cortical output within primates. These data seemingly align well with a recent analysis of neuronal complexity across primate species, indicating that larger brains (at least within the primates) tend to have increased neuronal complexity (Manger et al. 2013). These findings support the claim that the human brain is not only larger but also more complexly organized from both a neuronal and minicolumn perspective, in a manner that is expected due to allometric scaling at the microstructural level.


Fig. 5.2
The scaling of minicolumn width (using the pyramidal cell array) to brain mass as in a range of anthropoid primate species encompassing ~30 Ma of evolutionary diversification. Sources are the same as in Fig. 5.1 (Note that in certain cases an average minicolumn width had been computed by calculating the mean based on the minimum and maximum values reported in each study)
A survey of the literature reveals that minicolumn width appears to vary substantially in mammals among cortical regions and among species, and that brain size alone cannot account for the full range of variation. Figure 5.3 summarizes the current knowledge of minicolumns among species. Studies that utilized the apical dendrite bundle in investigations of minicolumn width within the occipital lobe show an interesting pattern, which coincides with what we already know about the visual cortex for some of these species. For instance, column width in the primary visual cortex of the rhesus monkey (Macaca mulatta) is on average smaller (23–30 μm) than that reported for rats (30–40 μm, average = 35 μm), rabbits (40–50 μm, average = 45 μm) and cats (55–60 μm, average = 57.5 μm; see Fig. 5.3). This is remarkable given the difference in brain size observed among these species for example the rhesus monkey has a brain size of 106.4 mm3 and the cat has a brain size of only 25.3 mm3 (Peters and Yilmaz 1993). This suggests that selection acting to increase column complexity, through for example, modifications in receptor and neurotransmitter types, may also serve as one route through which cortical output could be increased whilst maintaining or reducing overall column width (Buxhoeveden and Casanova 2002a, b). Gustafsson (1997) has proposed that while wide columns are likely to have more neurons and a greater synaptic weight, narrow columns may also offer greater discriminatory ability useful for feature detection. According to Peters and Yilmaz (1993) this apparent contradiction in the size of the macaque minicolumn was facilitated through the evolution of more complex, narrower cell columns that allow a more detailed specification of information. This pattern of selection for greater complexity within the macaque minicolumn is likely to be a synapomorphy, a derived feature shared with several other extant primate species. Livingstone and Hubel (1984) have shown that primates have indeed evolved specific modules in the primary visual cortex that are utilized for discriminating and processing form, color, and motion and that these differ markedly from that observed in rodents and felids. Other collaborative evidence has also indicated that the primate visual system in fact surpasses that of cats in the display of color and visual acuity (Orban 1984), lending support to the idea that primates are indeed endowed with more complex minicolumns. Without a detailed survey of the cortical column across primates, we can only postulate that the emergence of this evolutionary change in the visual system likely occurred about 35 Ma ago, when higher level color vision in the form of trichromacy evolved at the origin of the catarrhine clade and that this was selected to enhance the tracking and foraging of highly variable and seasonal food sources.
That evolutionary reductions in minicolumn width may be offset by an increase in minicolumn complexity, is highlighted by the available data within another group of large brained social species, the cetaceans. Morgane et al. (1988) have reported relatively small minicolumn sizes within the visual cortex of cetaceans (Stenella coeruleoalba and Tursiops truncatus), paired with descriptions of a discontinuous minicolumn structure across cortical layers (i.e., interruptions in the continuity of cellular columns through all cortical layers) (Morgane et al. 1988; Manger 2006). Glezer and Morgane (1990) have suggested that this unique adaptation in the cetacean cortical column is likely facilitated by the integration of column activity occurring through layer 1 which in cetaceans is specialized and contains around 70 % of the total cortical synapses. Interestingly, the minicolumns within the visual cortex of humans and dolphins also contain roughly the same number of synapses (Morgane et al. 1988), potentially explaining some of the similarities in cetacean and primate behavioral complexity (Marino et al. 2008) despite two very different structural arrangements. This illustrates the need for caution in oversimplifying measurements of cell column width as a direct correlate with functional output and highlights why a comparative perspective is important in helping to understand the range of variation in column structure within and across multiple species.
Investigations of homologous areas in other taxa are likely to yield new insight into species-specific differences in cortical column structure. For example, analyses of minicolumn morphology in the temporoparietal cortex (area Tpt) of humans, macaques and chimpanzees (Buxhoeveden and Casanova 2000) suggests that this cortical region within humans underwent substantial rearrangement, resulting in cortical columns in the left hemisphere reportedly 30 % wider than those observed in the left hemisphere of chimpanzees and macaques and also reportedly having more neuropil space than that observed in nonhuman primates examined thus far (Buxhoeveden and Casanova 2000; Buxhoeveden et al. 2001a, b). As summarized in Fig. 5.3, cortical column width measured in the temporoparietal cortex (area Tpt) is relatively uniform in the great apes and macaques, with humans possessing larger minicolumn sizes (50.4 μm). Such a restructuring in this extension of the human auditory cortex has been postulated to underlie our unique language abilities (Rilling et al. 2008). In addition, humans are also known to display significantly larger minicolumns in Broca’s area (Brodmann areas 44 and 45) than that observed in other great apes (chimpanzee, bonobo, gorilla and orangutan), adding further support to the claim for restructuring in these language circuits (Schenker et al. 2008). It is notable, however, that minicolumn widths in the temporal and parietal cortex are matched or exceeded in humans by squirrel monkeys, owl monkey, cats, and rats (Fig. 5.3). Such observations place claims of human uniqueness in a broad phylogenetic context and encourage re-evaluation of assumptions that modifications of minicolumn morphology is necessarily linked to the evolution of cognitive function.


Fig. 5.3
A summary of average minicolumn width by brain region in all species studied to date (Sources are the same as in Fig. 5.1)
Adaptive accounts of minicolumn variability among species often overlook the possibility that certain aspects of column structure could simultaneously remained unchanged or be subject to non-adaptive forces. A prime example of this is that reported in a reanalysis of morphometric variability in minicolumns of the primary visual cortex in humans, chimpanzees, and macaques, which confirmed the existence of species differences in minicolumn widths, but also indicated that the core columns space was relatively invariable across these taxa (Casanova et al. 2009). Without a comprehensive analysis of column core variability across several species using a common methodology it is difficult to determine whether conservation in the column core is specific to this clade (catarrhine primates) or whether it is indicative of broader primate or mammalian bounds for this structure. While the above examples and patterns are tentative given the paucity of data, they highlight the utility of a broad comparative approach for revealing evolutionary patterns of cortical column variability and how an analysis sensitive to variation and diversity could provide adaptive explanations that take into consideration the environments within which species evolved.
Evolution by natural selection operates by differential fitness among variable phenotypes within a population. There is evidence indicating that minicolumn widths do show continuous variation within a normal population and may be under the influence of multiple independent factors (Casanova 2006). Casanova et al (2007), have reported significant differences in minicolumn width and mean cell spacing of a control human group (n = 6) and that of three distinguished scientists suggesting that genetic, environmental and developmental factors (i.e., the duration of cell division cycle, number of founder cells, selective cell death cycle) are likely to have an impact on the column phenotype. Cumulatively, both intra- and inter-specific variation in cortical column morphology points in favor of an interpretation of the cortical phenotype, which is more strongly rooted in an evolutionary view of diversity.
Aside from size, minicolumns in different species may also show variability in their structural subcomponents (i.e., fibers and neurons). The following section is aimed at reviewing the evidence for phylogenetic differences indicative of diversity in the microcircuitry of mammalian cortical structure, with a focus on different subtypes of γ-aminobutyric acid (GABA)-containing interneurons, and implications for mental illness in humans.
5.3 Phylogenetic Diversity in GABAergic Interneurons and Their Relation to Minicolumns
Inhibitory GABAergic interneurons are a critical component of cortical microcircuitry and play a fundamental role in intra- and intercolumnar processing (Hendry 1987; DeFelipe 2002; Casanova et al. 2003; Buzsaki et al. 2004; Ascoli et al. 2008; DeFelipe et al. 2013). Interneuron subpopulations are also known to show significant phylogenetic variation both in the diversity and density of interneuron subtypes and in their distribution and patterns of development (e.g., Hof et al. 1999; Preuss and Coleman 2002; Hof and Sherwood 2005; Sherwood et al. 2007). Qualitative comparisons of inhibitory interneurons across taxa, have also revealed Order specific patterns, with primates displaying a greater proportion of inhibitory interneurons (i.e., greater than 20 %) in comparison to that observed in rodents (i.e., less than 15 %).(Hendry et al. 1987; Beaulieu et al. 1992; Gabbott and Bacon 1996; Gabbott et al. 1997; DeFelipe et al. 1999, 2002). Furthermore, studies of the development and migration of GABAergic cells have also observed an expansion of neurogenesis into the lateral ventricular neurepithelium of primates (Petanjek et al. 2009). These observations are indicative of the effects of phylogeny in the evolution of this system and its role in supporting the elaborate behavioral and cognitive attributes definitive of primates.
Inhibitory interneurons can be classified into subpopulations based on their immunoreactivity for the three calcium-binding proteins, calbindin (CB), calretinin (CR), and parvalbumin (PV) (Hendry et al. 1989; Glezer et al. 1993; DeFelipe 1997; Zaitsev et al. 2005) with each of these subpopulations playing in turn interacting with pyramidal cells to modulate processing within the cortical circuit. The subpopulations of CB- and CR-immunoreactive(ir) neurons are known to be involved in intracolumnar communication while interneurons immunoreactive for PV are involved in transcolumnar signaling.
An example of a phylogenetic pattern in GABAergic interneurons, is that observed for the primate cortex, where CB-ir double bouquet cells are known to contribute significantly to the morphology and distribution of minicolumns (Buxhoeveden and Casanova 2002b; Casanova et al. 2009). As a potential source for minicolumn diversity, we outline below the range of variation known for GABAergic neurons in mammals. Calcium-binding protein- expressing interneurons are known to vary significantly in their distribution between species, (Glezer et al. 1992, 1993; Hof et al. 1996, 1999 Glezer et al. 1998; Hof and Sherwood 2005; Zaitsev et al. 2005; Sherwood et al. 2007; Sherwood et al. 2009), while variation in GABAergic cell phenotype and density would be a consequence of evolutionary alterations in local microcircuit processing. A number of studies have indicated that the proportion of cortical interneurons also varies among species, with members of the order primates having a higher overall percentage relative to rodents, afrotheria, and xenarthrans; while the cetaceans markedly have the greatest proportion of cortical interneurons of all species studied to date (Hof et al. 2000; DeFelipe et al. 2002; Sherwood et al. 2009). Furthermore, studies have also indicated that variation in electrophysiological response properties may contribute to species level differences in GABAergic interneurons. While a study of PV-ir fast-spiking basket cells reported no significant morphological differences between macaque monkeys and rats (Povysheva et al. 2008), a significant difference in excitability of PV-ir basket cells was observed with the neurons of the macaque monkey having a higher input resistance and lower firing threshold than those of rats. This finding coincides with the lower frequency firing rates reported for rats in prefrontal cortical neurons (Povysheva et al. 2008) and is intriguing given that the actions of PV-ir interneurons appear to be essential for the accomplishment of working memory tasks (Rao et al. 1999) and other cognitive functions (Constantinidis et al. 2002).
In addition, phenotypic variation within interneuron subpopulations have also been reported between species. An example of this species level variation, is that reported between primates and canids, with chandelier cells in the primate primary motor and somatosensory cortex expressing PV-ir (DeFelipe et al. 1990), while this was not observed in canids (Hof et al. 1996). Furthermore, CB-ir double bouquet cells were not observed in the following groups: rodents, lagomorphs, artiodactyls xenarthrans, and afrotherians (Sherwood et al. 2009; Ballesteros-Yañez et al. 2005) but are reportedly present in primates (human and macaque monkey), and to a lesser extent carnivores (Ballesteros-Yañez et al. 2005). The connectivity of these double bouquet neurons, characterized by long descending bundles of axon collaterals that form columns targeting pyramidal cells within a very narrow space, is argued to represent a specialization of minicolumn inhibition within primates (del Rio and DeFelipe 1997a; DeFelipe et al. 2002, 2006; Ballesteros-Yañez et al. 2005). Its also worth noting that minicolumns within the human cortex are known to be comprised of double bouquet axon bundles associated with myelinated axons although not all minicolumns were associated with CB-ir double bouquet cells (Ballesteros-Yañez et al. 2005).
Several neuropathological abnormalities in humans have been noted that involve minicolumns and interneurons. For example, decreased numbers of CB-ir interneurons have been reported consistently in the prefrontal cortex of patients with schizophrenia (Beasley and Reynolds 1997; Reynolds et al. 2001; Beasley et al. 2002; Cotter et al. 2002; Eyles et al. 2002; Chance et al. 2005; Sakai et al. 2008) while CR-ir interneuron density is preserved (Woo et al. 1998; Reynolds and Beasley 2001; Zhang and Reynolds 2002). The relationship between PV-ir neurons distributions and schizophrenia is less clear, with reports of a decrease in density (Beasley and Reynolds 1997) or no change in density (Woo et al. 1997; Cotter et al. 2002). Alterations of minicolumn width (i.e., neuropil space) are also well-documented in schizophrenia (Reynolds et al. 2004; Casanova et al. 2005, 2008; Chance et al. 2005; Di Rosa et al. 2009). These changes in minicolumn morphology appear to be consistent with a developmental abnormality rather than a progressive pathological process (Casanova et al. 2005, 2008).
Alzheimer’s disease is also associated with a decrease of cortical CB-ir pyramidal neurons in some regions of human cortex (Ferrer et al. 1993; Nishiyama et al. 1993; Beasley et al. 2002) and has also been reported in the canine expression of dementia of the Alzheimer’s type (Pugliese et al. 2004) while both PV- and CR-ir neuronal subpopulations are spared (Ferrer et al. 1993; Hof et al. 1991; 1993; Pugliese et al. 2004). However, it should be noted that not all forms of dementia are associated with a reduction in cortical calcium-binding protein-containing interneurons (Hof et al. 1994; Gomez-Tortosa et al. 2001). The structure of minicolumns is selectively disrupted in Alzheimer’s disease, and the loss of columnar organization was related to the number of neurofibrillary tangles (Buldyrev et al. 2000). Tangles cluster into columns and their numbers are positively correlated with degree of cognitive loss in Alzheimer’s disease (Nagy et al. 1996). Minicolumn thinning was also noted in normal human aging and is a process that may be continuous with the increased risk of Alzheimer’s disease over the age of 65 (Nagy et al. 1996).
It has been postulated that cortical minicolumn GABAergic inhibitory control is also compromised in autism (Casanova et al. 2003). However, while dysregulation of the calcium-binding protein-ir interneuron populations was recently demonstrated within the hippocampus of patients with autism (Lawrence et al. 2010), comparable data are not available for neocortical regions. Nonetheless, autism and Asperger’s syndrome are associated with a narrowing of the minicolumns, specifically, the peripheral neuropil space (Casanova et al. 2002a, b, c, 2003). Because the peripheral neuropil space is dependent upon inhibitory interneuron populations, a deficit in GABAergic control is suspected. In particular, the modulation of minicolumnar activity would be altered for both local and long-range connectivity, resulting in collateral over-excitation among minicolumns (Casanova 2008; Casanova and Trippe 2009). Such a deficit is also suspected to contribute to the incidence of seizures in autistic individuals (Casanova et al. 2003). This relationship finds support in recent reports of deficits in both PV- and CR-ir interneurons with focal cortical dysplasias associated with epilepsy (Zamecnik et al. 2006; Barinka et al. 2009).
5.4 Conclusions
Variability not only in the connectivity of the minicolumn but also in the subtle subcomponents of the columnar organization such as composition of interneuron subtypes, are a primary source of inter and intraspecific differences. The comparative approach highlights the need to go beyond the restraints enforced by a narrow view of the cortical minicolumn and challengers us to embrace diversity in our interpretations of minicolumn phenotype. It is only through the lens of variation that we are able to bring together discussions of the minicolumn with the evolutionary framework that unites the biological sciences.
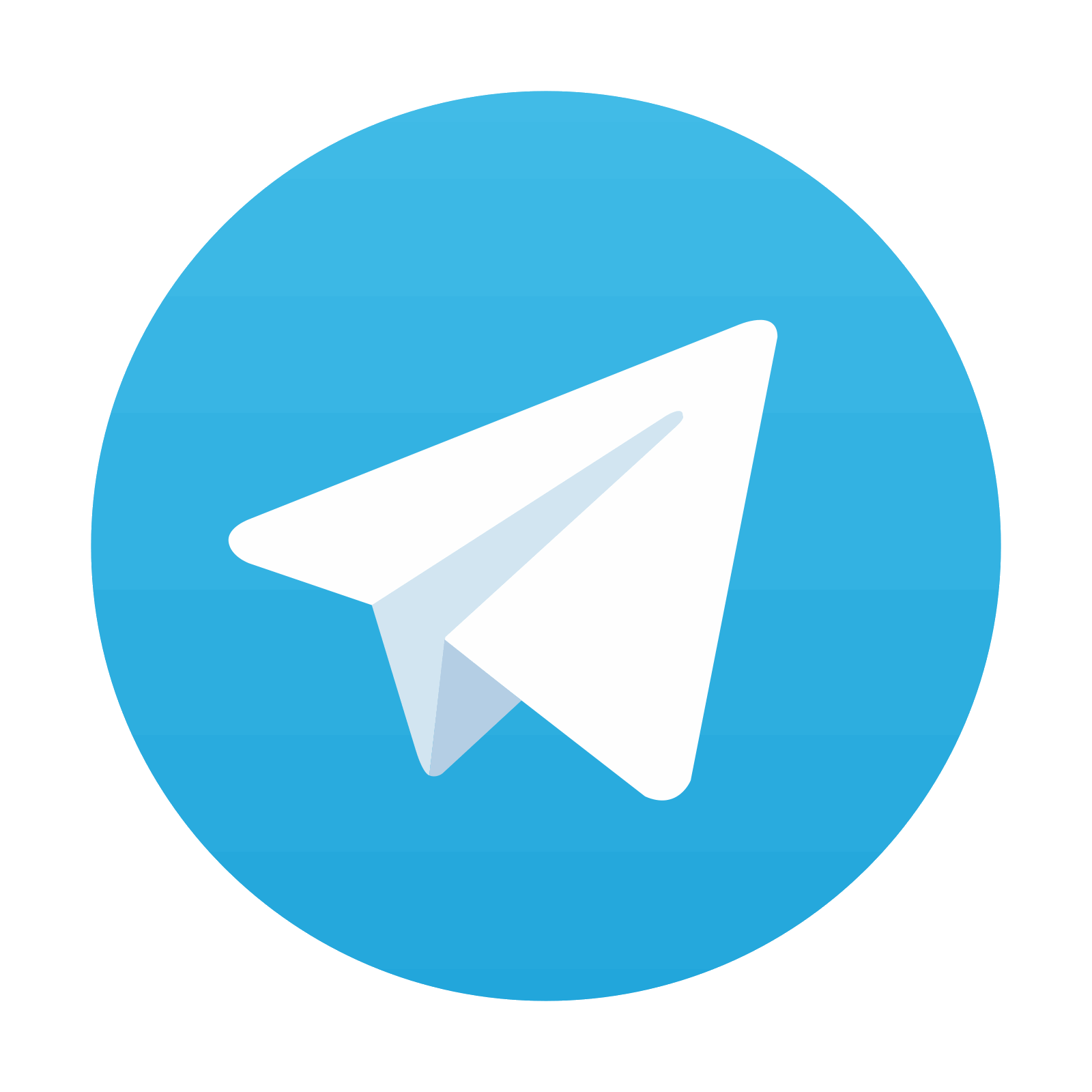
Stay updated, free articles. Join our Telegram channel
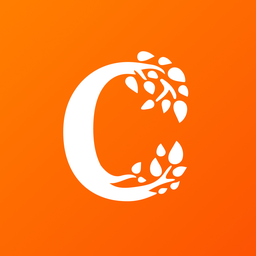
Full access? Get Clinical Tree
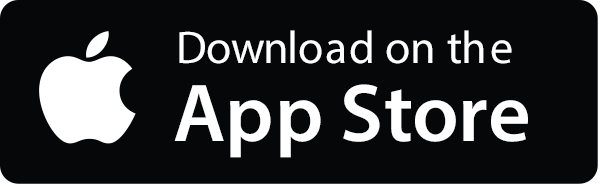
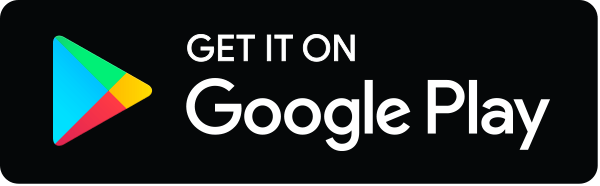