The cellular origin of glioblastoma is complex. Despite decades of research, the understanding of the cellular, molecular, and pathogenetic architecture of this lethal disease is still evolving. Other chapters discuss the epidemiology, risk factors, associated clinical outcomes, and molecular targeting for glioblastoma. There are also chapters that describe the mechanisms of small molecule inhibitors, targets for therapeutics and their molecular basis, immune evasion, and mechanisms of angiogenesis and proliferation. This chapter provides a practical and concise overview for practicing clinicians of the well-understood and best-studied mechanisms that cause an innocuous glial cell to transform into glioblastoma.
Classification and histology
The most commonly used and widely accepted classification schema for malignant gliomas is based on a consensus by the World Health Organization (WHO), first published in 1979, updated in 2007, which designated glioblastoma a WHO grade IV neoplasm. WHO grading was based on glioblastoma as a “cytologically malignant, mitotically active, necrosis-prone neoplasm typically associated with rapid pre- and postoperative disease evolution and fatal outcome.” Deficiencies with the use of the WHO schema include inability to account for molecular subtypes, therapeutic responses, or size and location of lesion. This shortcoming has led many scientists and clinicians to advocate for a molecular grading system that more accurately takes into account the evolving understanding of the molecular pathogenesis of glioblastoma. Some of which is outlined in the following chapter.
On histology, gliomas show several characteristic findings. These findings include anaplasia, high levels of mitotic activity, cellular pleomorphism, nuclear atypia, and coagulation necrosis that accompanies microvascular proliferation. Necrosis is the result of ischemia caused by endothelial cell hyperplasia and hypertrophy. There is a hypercellularity present throughout the tumor, with atypical mitotic figures, bizarre nuclei, and multinucleated cells. Commonly referred to is the concept of pseudopalisading necrosis, which consists of tumor cells that appear to surround centralized necrotic zones. The presence of both necrosis and endothelial proliferation is necessary for the histologic diagnosis to be complete, to differentiate glioblastoma from anaplastic astrocytoma ( Fig. 3.1 ).

These histologic findings have been in use for decades, and remain important in the initial classification after biopsy or resection of a tumor. Advances in modern molecular and genetic techniques, including large-scale profiling of data sets both at the national level (The Cancer Genome Atlas) and the international level (International Cancer Genome Consortium), have resulted in a more expansive and complex understanding of the immense heterogeneity found within tumors classified as glioblastoma ( Figs. 3.2 and 3.3 ).


Recently, and importantly, Verhaak and colleagues described a classification of high-grade gliomas into 4 distinct types according to genetic and molecular features based on genomic abnormalities found in The Cancer Genome Atlas Network. The investigators used the factor analysis method to integrate data from glioblastoma and normal brain samples on 3 different gene expression platforms. Consensus clustering and further validation ultimately yielded 4 subtypes that were named from their signature gene expression: proneural, neural, classic, and mesenchymal.
The classic subtype consisted of chromosome 7 amplifications paired with chromosome 10 loss 100% of the time. In addition, high-level epidermal growth factor receptor ( EGFR ) amplification was found in 97% of the classic subtype, and infrequently in others. There was also a lack of TP53 mutations despite TP53 being a frequently mutated gene in glioblastoma. In the mesenchymal subtype, there were focal hemizygous deletions at region 17q11.2, which contained the gene NF1 , and there were frequent comutations of NF1 and PTEN that intersected the AKT (protein kinase B) pathway. The proneural subtype was defined mostly by alterations of PDGFRA and IDH1 , and most TP53 mutations were found in the proneural subtype. The neural subtype had expression of several different neuronal markers that were ontologically consistent with genes involved in axonal and synaptic transmission as well as neuronal projection ( Fig. 3.4 ).

After classification of subtypes was completed, the effect of level of treatment intensity was evaluated for each of the subtypes. Aggressive treatments reduced mortality in the classic, mesenchymal, and neural subtypes (trend toward, but not statistically significant), but not the proneural subtype ( Table 3.1 ).
Classification | Characteristics |
---|---|
Classic | Chromosome 7 amplification Chromosome 10 loss EGFR amplifications Lack of TP53, NF1, PGFRA, or IDH1 mutations Expression of NES, Notch and Sonic hedgehog signaling pathways |
Mesenchymal | NF1 deletions/mutations PTEN mutation Expression of mesenchymal markers (CHI3L1, MET) Genes of tumor necrosis factor family and NF-kB pathways |
Proneural | PDGFRA alterations IDH1 mutations TP53 mutations Expression of development genes (PDGFRA, NKX2-2, OLIG2, SOX, DCX, DLL3, ASCL1, TCF4) Developmental disturbances |
Neural | Overexpression of neuronal markers (NEFL, GABRA1, SYT1, SLC12A5) Neuron projection and axon and synaptic transmission disturbances |
Primary and Secondary Glioblastoma
It has been recognized since the 1940s that 2 distinct clinical histories exist for glioblastoma. In the first, there is a rapidly progressive onset of disease without a preexisting low-grade tumor, occurring most frequently with older patients. In the second, there is the transformation of an existing low-grade tumor to glioblastoma in a younger patient and a comparable clinical course. These two pathways have been evaluated and characterized by their molecular genetics and are referred to respectively as primary and secondary glioblastoma.
The ability to differentiate between these 2 different types of glioblastoma progression is based on significant genetic and epidemiologic evidence. First and foremost, only approximately 5% of all cases of glioblastoma are thought to be manifestations of secondary glioblastoma, an observation based on several population-based studies, including a landmark study by Ohgaki and colleagues that examined 715 glioblastomas in Zürich, Switzerland, between 1980 and 1994. The study also examined age differences between diagnostic groups and found that the mean age of primary glioblastoma was 62 years, compared with a much younger 45 years for patients with secondary glioblastoma.
The molecular and genetic pathways to both primary and secondary glioblastoma also provide the opportunity to discuss the wayward progression of an astrocyte or precursor cell to a high-grade glioma ( Fig. 3.5 ).

TP53 and MDM2
TP53 is a gene found on chromosome 17 that codes for a 53-kDa protein involved in various aspects of the cell cycle, including cell death, response of cells to DNA damage, differentiation, and vascular phenomena. It is generally thought that TP53 mutations are found more frequently in secondary glioblastoma because they are often the first detectable genetic alteration in up to two-thirds of low-grade astrocytomas, although they may occur in up to 30% of primary glioblastomas as well given the variety of functions enacted by TP53 in the cell cycle. After DNA damage, p53 is activated and induces a variety of genes, including p21, which is a cycle-independent kinase inhibitor. After induction of p21 , MDM2 (mouse double minute 2 homolog, an E3 ubiquitin-protein ligase whose key target is the p53 tumor suppressor) becomes involved in an autoregulatory feedback loop. MDM2 is able to repress p53 by binding to and blocking the N-terminal transactivation domain of p53. MDM2 binds to both the mutant and wild-type TP53 proteins, inhibiting transcription of activation of wild-type TP53 . As a component of this feedback loop, transcription of the MDM2 gene is induced by the wild-type TP53 . As a result, the normal feedback loop, which would regulate the activity of the TP53 protein and expression of MDM2 , is disrupted. Also involved in this loop is the role of a p14 gene product, which binds to MDM2 and inhibits MDM2 -mediated TP53 silencing and degradation. As a result, loss of normal TP53 function could follow altered expression from TP53 itself, MDM2 , or p14 ( Fig. 3.6 ).
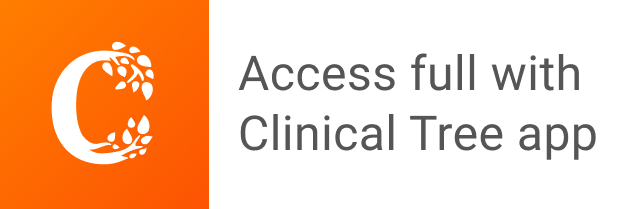