Introduction
Multiple treatment modalities are effective for major depressive disorder (MDD), including medications, certain psychotherapies, and brain stimulation approaches such as electroconvulsive therapy (ECT) or repetitive transcranial magnetic stimulation (rTMS). However, treatment resistance, that is lack of full symptom remission after one or more rounds of antidepressant treatments of adequate dose and duration, is the rule, not the exception with MDD ( ). For example, the NIMH-supported Sequenced Treatment Alternatives to Relieve Depression (STAR*D) study was a large trial that treated depressed patients across four treatment steps, beginning with citalopram monotherapy ( ). Only about one-third of patients remitted with initial treatment with citalopram, and another third to second-level treatments with either monotherapies, combinations of medications, or combined citalopram and cognitive behavioral therapy. By the third and fourth steps, only 13.7% and 13% of patients remitted, respectively. The cumulative remission rate was only 67%, and over half of remitted patients relapsed during a 12-month naturalistic follow-up phase ( ). Clearly, something is preventing response in many people and causing others to relapse. The purpose of this chapter will be to address the neurobiological impediments to antidepressant treatment response.
Why do people get depressed?
Allostasis is the normal physiological adaptation to maintain homeostasis in face of stressors ( ; ). The cumulative effect of stress on the body is referred to as allostatic load ( ). The responses to threats of homeostasis, such as activation of the autonomic nervous system and the hypothalamic–pituitary–adrenal axis, and cytokine responses are adaptive in the short-term ( ). In fact, short-term stressors that are perceived to be controllable have a positive effect on brain health, enhancing cognitive and physical performance ( ). However, chronic allostatic loading is harmful and is associated with a range of conditions, including abdominal obesity, hypertension, diabetes, cardiovascular disease, arthritis, and depressive symptoms ( ; ; ). Persistent activation of endocrine, cardiovascular, metabolic, and emotional responses increases risk for and progression of disease ( ). Brain allostatic loading is a known factor in depression ( ). For example, found that several aspects of allostatic responses, including HPA activity, immune response, anabolic activity, and cardiovascular disease, were associated with depressive symptoms, after adjusting for demographic, socioeconomic, and other health-related factors in older adults. How, then, does that lead to depression?
Much work over the last two decades has focused on the dynamic regulation of neuronal dendrites, spines, and synapses in brain, collectively referred to as neuroplasticity. Spines are protrusions from dendrites that form synapses with other cells ( Fig. 4.1 ). A single neuron can have thousands of spines and synapses. These change very dynamically in response to environmental cues. The formation, maintenance, and regression of spines and synapses is regulated by a wide range of factors; chief among these are neurotrophins such as brain derived neurotrophic factor (BDNF) and related trophic factors such as insulin-like growth factor-1 (IGF-1) and vascular endothelial growth factor (VEGF) ( ). Interestingly, acute stress that is short-term and controllable actually increases BDNF along with spines and synapses ( ). However, chronic, unpredictable, and uncontrollable stress reduces BDNF synthesis and release and regression of dendrites and spines, a process that is associated with depression-like behavior in animal models ( ).

The effects of stress on the brain are complex and vary by region. For example, stress and glucocorticoids result in regression of dendrites and loss of dendritic spines and synapses in hippocampus and frontal cortex ( ). Conversely, acute stress increases spines, and chronic stress leads to enlargement of dendrites in the basolateral amygdala ( ). However, the net loss of synapses in frontal cortex and hippocampus, particularly those connecting pyramidal and other neurons with interneurons, appears to underlie depression.
The process of removal of synapses, spines, and dendrites is a type of macroautophagy, usually just referred to as autophagy, which is an adaptive process by which cells remove components. A structure called a phagophore engulfs cellular material forming an autophagosome, which merges with a lysosome, resulting in the degrading of proteins. Various types of cell stress, including toxins, microorganisms, metabolic stress, and ischemia, activate autophagy, resulting in the clearing of damaged proteins or cell remodeling ( ). This process is tonically suppressed by the mammalian (or mechanistic) target of rapamycin complex 1 (mTORC1), discussed in greater detail below. A variety of metabolic factors can suppress mTORC1 activity, releasing the autophagic process and leading to loss of spines and synapses ( ). Therefore, maintaining mTORC1 activity is critical in preserving spines and synapses.
Interneurons serve as functional regulators of pyramidal and related neurons (referred to as principal neurons), which mediate communication both within and between brain regions. Some types of interneurons release gamma-aminobutyric acid (GABA), the principal inhibitory transmitter in the nervous system, which functions as a key regulator of brain activity by “gating” both input and output of principal neurons ( ). Glutamate is the main excitatory output transmitter of principal neurons, which is balanced by GABA. Chronic stress and the depressed state that results from stress involve loss of GABA synapses on principal neurons and an imbalance in input and output. This excitatory (glutamate) to inhibitory (GABA) imbalance occurs because of a reduction in principal neuron dendrites, spines, and synapses ( ; ). A result of stress is a loss of inhibitory control by GABA interneurons.
BDNF and other trophic factors increase dendritic volume and density, and lead to the formation of spines and synapses. It also induces cell replication of neurons in the hippocampus (neurogenesis) ( ). However, continued input by BDNF is also required for the maintenance of dendrites, spines, and synapses. Chronic stress and the resulting depression-like behaviors in rodents are associated with reduced synthesis and release of BDNF, which results in progressive loss of spines and synapses ( ). Antidepressant treatments appear to reverse this process and increase BDNF activity. The binding of BDNF to TrkB initiates a cascade of intracellular signaling molecules that ultimately activate mTORC1 ( Figs. 4.1–4.3 ) ( ). The complex consists of the mTOR protein itself, along with regulatory-associated protein of mTOR (Raptor), and mammalian lethal with SEC13 protein 8 (nLST8). Proline-rich AKT1 substrate 40 kDa (PRAS40) interacts and activates mTORC1 following phosphorylation by RAC-alpha serine/threonine-protein kinase (AKT). AKT also inhibits the TSC1/2 protein complex and inhibitor of mTORC1 ( Fig. 4.3 ). mTORC1 regulates the synthesis of proteins, including those that form spines and synapses ( ). Stress inhibits this process in part by increasing cortisol which binds to the glucocorticoid receptor (GR) and inhibits mTORC1 ( ). Under normal circumstances, mTORC1 counterbalances this by inhibition of GR gene transcription. However, high or chronic stress unbalances this system resulting in reduced mTORC1 activity. Activation of the BDNF–TrkB–mTORC1 pathway leads to the genesis and maintenance of dendrites, spines, and synapses. Stress counters this by inhibition of both BDNF and mTORC1. As we will see below, antidepressant treatments help to restore BDNF and mTORC1 activity, leading to normalization of dendrites, spines, and synapses ( Fig. 4.2 ).


How do antidepressant treatments work?
Antidepressant treatments work by widely divergent proximal mechanisms—contrast, for example, the effects of selective serotonin (5-HT) reuptake inhibitors (SSRIs) with electroconvulsive therapy (ECT) or repetitive transcranial magnetic stimulation (rTMS). However, the net effect of most or possibly all effective antidepressant treatments is to increase the activity of trophic factors, particularly BDNF and its receptor tyrosine receptor kinase B (TrkB) (also known as tropomyosin receptor kinase B) ( ; ). Direct injections of BDNF and other trophins like neurotrophin- 3 have antidepressant-like effects in animal models of depression when injected directly into the brain ( ). Inhibition of BDNF ( ), TrkB ( ), or mTORC1 ( ) as well as other trophins like IGF-1 ( ) and VEGF ( ) block the effects of antidepressant treatments. Moreover, a series of studies indicated that depressed patients have lower BDNF levels prior to treatments, which increase after treatment ( ). However, antidepressant medications such as SSRIs do not directly affect these factors. How, then, do they produce effects on trophins and related molecules?
Fig. 4.2 illustrates the complex mechanisms of action of standard antidepressant treatments, focusing on the actions of SSRIs. Tryptophan (TRP) is taken up into the presynaptic neuron and is converted to 5-hydroxytryptophan (5-HTP) by tryptophan hydroxylase (TPH) (primarily TPH2 in brain). 5-HTP is converted to 5-HT by aromatic amino acid decarboxylase (AAAD). Free 5-HT is taken up into presynaptic vesicles by vesicular monoamine transporter 2 (VMAT2). The vesicles merge with the plasma membrane, releasing 5-HT into the synapse. There is a similar process with norepinephrine (NE), which is required by many antidepressant drugs. The NE precursor amino acid tyrosine is taken up by the presynaptic neuron and converted initially to l -DOPA by tyrosine hydroxylase (TH), then to dopamine (DA) by AAAD, and finally to NE by dopamine beta hydroxylase (DBH) (Fig. 4.2) . The vesicles merge with the plasma membrane, releasing 5-HT into the synapse. There is a similar process with norepinephrine (NE), which is required by many antidepressant drugs. The NE precursor amino acid tyrosine is taken up by the presynaptic neuron and converted initially to L-DOPA by tyrosine hydroxylase (TH), then to dopamine (DA) by AAAD, and finally to NE by dopamine beta hydroxylase (DBH). DA and NE are also taken up into vesicles by VMAT2 and released into the synapse.
5-HT binds to receptors that activate adenylate cyclase, which converts adenosine triphosphate (ATP) to cyclic adenosine monophosphate (cyclic AMP) through several 5-HT receptors (5-HT 4 , 5-HT 6 , and 5-HT 7 ). SSRIs block 5-HT reuptake, which increases 5-HT interactions with postsynaptic receptors (Fig. 4.2) . Activation of the 5-HT receptor leads to coupling with a guanine nucleotide-binding protein (g-protein) which, in turn activates adenylate cyclase, producing cyclic AMP. The latter then interacts with protein kinase A (PKA), which releases its catalytic subunit (C), which phosphorylates the transcriptional factor cyclic AMP response element binding protein (CREB). Similar downstream effects can occur with 5-HT binding to receptors that activate phospholipase-C (PLC) ( ) (e.g., the 5-HT 2 family of receptors), which catalyzes the conversion of phosphatidylinositol 4,5-bisphosphate (PIP2) to inositol triphosphate (IP3) and diacylglycerol (DAG). The latter activates protein kinase C, which phosphorylates CREB. Drugs that increase synaptic NE have similar actions via NE beta-2 receptors that are also positively coupled to AC ( ). Phosphorylated CREB then translocates to the nucleus where it binds to a DNA enhancer region called a cyclic AMP response element (CRE) in the promoters of specific genes. This increases the transcription of a wide range of genes, including those coding for BDNF, TrkB ( ; ; ), and VEGF ( ). This system is also affected by other trophins such as NT-3 ( ) and IGF-1, which increase CREB phosphorylation ( ). The downstream effects of antidepressants on BDNF and other trophic factors appear to be required to normalize the reduced dendrites, spines, and synapses in the depressed brain and restore normal mood ( ). Other effective antidepressant treatments such as ECT ( ) and rTMS ( ) also affect BDNF, TrkB, and other trophic elements.
Cells maintain homeostasis through the balance of activating and inhibiting processes. While the effects described above are occurring, there are counteracting elements that dampen cell signaling. For example, when NE or 5-HT transporters are blocked, the transmitters not only bind to postsynaptic receptors, but also to presynaptic autoreceptors: NE alpha-1, and 5-HT 1A (5-HT 1A ) and 1B (5-HT 1B ) receptors ( ). These inhibit the release of NE and 5-HT respectively. Although these receptors do eventually downregulate with time, they slow the actions of antidepressants. Blier and colleagues ( ) conducted a series of experiments, first in rodents and then in human with the 5-HT 1A antagonist pindolol showing that it prevented the acute reduction in 5-HT release seen after short-term administration of SSRIs and accelerated the antidepressant response. The postsynaptic receptors coupled to the effector mechanisms described above (e.g., adenylate cyclase) downregulate after repeated activation by neurotransmitters by internalization (called receptor trafficking), making them vulnerable to degrading enzymes ( ), and by uncoupling from g-proteins. In addition, there are a large number of enzymes within neurons that metabolize or inhibit the elements described earlier. For example, there are enzymes that degrade drugs, proteins, and transmitters. Others inhibit protein action through the process of either phosphorylation (i.e., adding a phosphoryl group [P + O 3 2 − ]) at serine, threonine, or tyrosine amino acids in proteins, or dephosphorylation. A well-known example of the latter is the inactivation of cyclic AMP by phosphodiesterases, but there are many other instances. These compensatory mechanisms maintain homeostasis in the cell but work against the mechanisms of action of standard antidepressants.
There are mechanisms of action of standard antidepressants that extend beyond uptake blockade. These effects include acting as antagonists of 5-HT 2A (mirtazapine, trazodone, nefazodone, and the atypical antipsychotics), 5-HT 3 (mirtazapine, vortioxetine, and the atypical antipsychotics clozapine and olanzapine), 5-HT 7 (vortioxetine) receptors, and acting as partial agonists (nefazodone, buspirone, vilazodone, and the atypical antipsychotics aripiprazole, asenapine, brexpiprazole, cariprazine, clozapine, and ziprasidone) or full agonists (vortioxetine) of 5-HT 1A . These effects confer anxiolytic- and antidepressant-like effects in animal models. Some of these effects appear to be direct, perhaps not involving neurotrophic actions. For example, acute activation of 5-HT 1A , or blockade of 5-HT 2A in the amygdala produces robust anxiolytic effects. In other instances, the effects are similar to the ones described for uptake inhibitors earlier. For example, activation of 5-HT 2A receptors inhibits BDNF mRNA synthesis, which is reversed with 5-HT 2A selective antagonists ( ). Therefore, some of the antidepressant effects of these mechanisms may depend on a downstream neurotrophic response.
Why do antidepressants fail?
Psychological and social factors
Fig. 4.2 illustrates some of the factors involved in determining if someone will respond to a medication or not. Beginning with the inset at the upper left—there are patient-level factors that affect response that have nothing to do with physiology. Could the patient afford the medication? Did they actually take it consistently? Did they continue to take it long enough for it to work? Could they tolerate any side effects that occurred? A third or more of patients will discontinue an antidepressant within the first 10 weeks of treatment ( ). This increases to two-thirds or higher by 6 months ( ). Early termination of medication occurs often in patients without consulting their health care professional. Collectively, these patient factors are the most common reason for antidepressant treatment failure.
There are also clinician factors that influence outcome. The relationship between prescriber and patient, referred to as the therapeutic alliance, is an important part of the outcome because it affects the patient’s therapeutic engagement and adherence to treatment. As many as one-third of prescriptions are never filled, and half of medications taken chronically are not taken as prescribed ( ). Clinicians are sometimes not thoughtful in prescribing antidepressants. Some use only a single, starting dose of a medication and do not titrate based on response; this is unlike treatments for other diseases like hypertension or diabetes in which regular measurement of outcomes is done and treatment adjusted accordingly. This is related in part to the fact that for antidepressant treatment, outcome is not usually measured ( ). Prescribers also often do not take mechanism of action into consideration when selecting a medication. For example, is a third SSRI likely to be helpful when the first two weren’t? Finally, psychotherapy for depression is seldom evidence-based ( ; ). The clinician impacts the outcome of treatment almost as much as the patient.
Non-CNS physiological causes
There are physiological reasons outside of the brain for why a medication does not work. For example, is the medication adequately absorbed? The absorption of an orally-administered drug depends on a range of factors, including residence time in the intestine and gut pH ( ). Some drugs are absorbed better when taken with a meal containing fat, but the absorption rate is slowed for most drugs taken with food. Once absorbed, all antidepressant drugs undergo first-pass (and subsequent) metabolism. Many antidepressants are metabolized by cytochrome enzymes, which vary widely between individuals, ranging from poor to ultrarapid metabolism ( ). This is particularly true for two enzymes that metabolize many psychotropics: cytochrome P450 (CYP) 2C19 and CYP2D6 ( ). This is why doses range so widely, and why recommended doses only represent guidelines, not absolutes. Venlafaxine, for example, is a CYP2D6 substrate. The enzyme activity can range from poor to ultrarapid metabolizer status. This means that some people will not be able to tolerate even the lowest available dose, 37.5 mg, while others never achieve an adequate blood level at 375 mg. Further, most drugs are metabolized by several CYP enzymes, which expand the possibility of CYP enzyme involvement in nonresponse. Prescribers should be aware of the various factors influencing drug absorption, metabolism, distribution, and elimination.
Neuronal and related cellular causes
Returning to Fig. 4.2 : There is an enormous number of factors that must be working adequately for any antidepressant treatment to work. We could even say that it is surprising that antidepressants work at all, given the number of factors that influence the outcome. This is why it is hard to implicate any single factor in influencing the outcome of antidepressant treatments. For example, some, but not all studies have found positive but very modest associations of polymorphic variants of the serotonin transporter gene (which goes by the designation SLC6A4 ) and outcome of serotonin reuptake inhibitor treatment ( ). The most common polymorphic variant involves a region in the gene promoter referred to as the serotonin-transporter-linked polymorphic region (5-HTTLPR) ( ). This is a variable number tandem repeat region of the promoter with two common forms, short and long, referring to the number of repeated segments. The long variant is more active and is associated with a higher level of gene transcription and, therefore, more of the transporter. This is also a common G/A variant of the long allele, commonly expressed as L G and L A ; the G variant of the long allele is also associated with reduced transcription. It has been hypothesized in the past that the variants associated with reduced availability of the serotonin transporter may reduce the effectiveness of serotonin reuptake inhibitors (SRIs). In fact, while this is true of most studies, the association of 5-HTTLPR variants and clinical outcome has been very weak. However, this is not surprising because antidepressants could fail for many other reasons in people who do not carry the loss of function 5-HTTLPR or other SLC6A4 variants, weakening the associations.
Beginning from the left in Fig. 4.2 , tryptophan (TRP) is the precursor of serotonin, which is of course required for the actions of the SSRIs. TRP is an essential amino acid in humans and is derived exclusively from dietary sources. TRP is transported across the blood–brain barrier (BBB) by a carrier protein that also transports isoleucine, leucine, phenylalanine, tyrosine, and valine ( ). Competition for this carrier limits the amount of TRP that enters the brain, and, therefore, the amount of 5-HT that can be made. The actions of SSRIs are sensitive to TRP levels in the body. Delgado et al. showed that the antidepressant benefits in depressed patients who had responded to SSRIs could be rapidly reversed by depletion of TRP ( ). This procedure consisted of administering a TRP-free amino acid mixture, which was hypothesized to be lead rapidly to protein synthesis peripherally, which, then, results in a 70%–80% depletion of plasma TRP ( ). This temporarily reversed the actions of SSRIs within hours, which recovered after giving TRP. These findings suggest that SSRI antidepressant response is sensitive to TRP levels.
Why would the action of SSRIs be so sensitive to TRP levels? In a typical physiological state, most of the 5-HT that is released into the synapse is taken by up by serotonin transporters and stored for future use in presynaptic vesicles. There is, of course, some loss in this process requiring new synthesis of 5-HT. However, the system is relatively efficient in conserving 5-HT. When a 5-HT uptake inhibitor is given, this process is disrupted, and it appears that most of the 5-HT is not taken up. Kinetic studies have shown that a 5-HT transporter deficiency (which mimics the SSRI effect) significantly reduces reuptake of 5-HT. Mice lacking one copy of the 5-HT transporter gene (i.e., heterozygous, +/), a kind of physiological imitation of the effects of SSRIs, show substantial reductions in the rate and total amount of 5-HT reuptake ( ). In addition, when an SSRI is given chronically to TPH2-deficient mice, in which 5-HT synthesis is reduced by 80%, there is a dramatic reduction in neuronal serotonin of about 99%! ( ) By contrast, the control mice without the TPH2 reduction showed no depletion after SSRI administration. Therefore, 5-HT has to be continuously synthesized for SSRIs to work. Therefore, TRP availability and conversion to 5-HT are important determinants of SRI response. In addition, the speed at which relapse occurs after TRP depletion shows that the effect of an SSRI is very “fragile”—that is, it can be reversed by a short-term reduction in 5-HT availability. A similar effect is seen with the depletion of the NE precursor tyrosine on the response to NE reuptake inhibitors in some studies ( ; ). However, the effect may be somewhat less robust than with TRP depletion ( ; ) and study results have been inconsistent ( ; ).
Are there natural physiological equivalents to TRP depletion? One possible set of mechanisms is systemic inflammation that occurs in inflammatory diseases such as rheumatoid arthritis or lupus erythematosus but is also associated with obesity and metabolic diseases like type 2 diabetes ( ). Systemic inflammatory diseases are known risk factors for depression and both inflammation and obesity reduce the response to SSRIs ( ). Inflammation depletes TRP and 5-HT and reduces synaptic 5-HT by several processes ( Fig. 4.4 ). The upper panel of Fig. 4.4 illustrates two different mechanisms for reduced 5-HT synaptic signaling. A variety of inflammatory factors activate the enzyme indoleamine-2,3-dioxygenase (IDO), which converts TRP to kynurenine ( ). Persistent IDO activation leads to TRP and 5-HT depletion ( ), theoretically reducing response to antidepressants. Interestingly, functional single-nucleotide polymorphisms (SNPs) of the IDO1 gene are associated with antidepressant response ( ). A second pathway specifically affects synaptic 5-HT levels. Tumor necrosis factor alpha (TNF) and other inflammatory mediators activate the enzyme P38 MAP kinase (P38 MAPK) ( Fig. 4.4 ). This in turn phosphorylates the serotonin transporter (5-HTT), which increases the rate of 5-HT reuptake into the presynaptic neuron ( ). These mechanisms and others like chronic systemic inflammation can lead to reduced 5-HT signaling and response to serotonergic antidepressants.
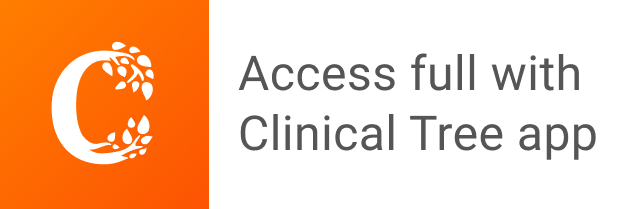