Fig. 12.1
Averaged spine densities on pyramidal cell apical dendrites as a function of distance from the cell body, cortical region, and cortical layer for both ASD and control subjects. Error bars indicate the standard error of the mean for each value. ASD subjects showed consistently elevated values within superficial layer II of parietal (BA 7), frontal (BA 9) and temporal (BA 21) regions (Reprinted from Hutsler and Zhang (2010) with permission from Elsevier)
Although the axonal content of the corpus callosum has not been directly examined in ASD, postmortem studies of frontal lobe white matter have shown both alterations to myelin and changes in axon populations (Zikopoulos and Barbas 2010). These studies point directly to reductions in the largest myelinated fibers, which likely correspond to long-range connections. Although further studies of the microanatomy of the white matter in ASD are needed to fully evaluate the potential structural bases of functional underconnectivity, the corpus callosum (CC) provides an ideal location to examine this issue because of its predictable fiber orientation, topographic organization, and lack of axons that form local connections.
12.2 Overconnectivity in ASD
While long-range functional underconnectivity has received a great deal of focus in ASD, it has also been suggested that ASD individuals may demonstrate short-range overconnectivity (Courchesne and Pierce 2005; Hughes 2007). Structural imaging studies have reported an increased volume in white matter compartments associated with short-range connections (Herbert et al. 2003, 2004).
In addition, this type of connectional change has been proposed to co-occur alongside of deficiencies in long-range cortico-cortical connections in ASD and include increases in short-range connections, as well as increased connections between subcortical areas and the cortex (Mizuno et al. 2006). This pattern of connectional change is partially supported by functional imaging studies showing a disconnection between distant cortical regions and studies demonstrating increased activity in regions associated with early cortical processing (Just et al. 2004; Koshino et al. 2005). It has been proposed that this connectional architecture may result in the absence of top-down modulation of early sensory processing (Frith 2004) and an inability to integrate spatially distant information (Frith 2004; Just et al. 2004). In addition, local overconnectivity has also been tied to improvements in processing local information at the expense of global structure (Baron-Cohen and Belmonte 2005) and theories of weak central coherence (Frith and Happé 1994).
The spines that cover the dendritic arbors of pyramidal cells in mammals are thought to support excitatory connections and have been widely used as an index of presynaptic excitatory connectivity onto these neurons in both animal and human studies (Fiala et al. 2002). During human development, dendritic spines first appear on neurons within the deep cortical layers in the late prenatal period (Huttenlocher and Dabholkar 1997; Michel and Garey 1984). In contrast, neurons in the superficial layers do not express spines until several months postnatally (Koenderink and Uylings 1995; Koenderink et al. 1994). Spine density reaches a maximum between 12 and 36 months postnatal depending upon the cortical location (Huttenlocher and Dabholkar 1997; Michel and Garey 1984). This maximum is followed by a gradual decline in spine numbers, which is believed to be associated with the culling of unused connections and the establishment of mature cortical networks. Since spine culling occurs postnatally, this process is also widely thought to be experience dependent and guided by environmental interactions (Huttenlocher and Dabholkar 1997). Previously, it has been proposed that individuals with ASD may have impaired synapse elimination attributable to their inability to fully utilize this available environmental input (Courchesne 2004; Frith 2004; Mundy and Neal 2001). This hypothesis is very similar to one proposed for fragile X syndrome, another pervasive developmental disorder where over-expression of synaptic spines has been demonstrated using Golgi methods in postmortem human tissue (Irwin et al. 2000, 2001; Sabaratnam 2000).
In ASD, we examined synaptic spines on pyramidal neurons located within the superficial and deep cortical layers of frontal, temporal, and parietal eulaminate isocortex (Brodmann’s Areas 7, 9, and 21; Hutsler and Zhang 2010). Spine densities were averaged across the distance from the cell body to directly compare three cortical layers (II, III, and V), and three morphological types of dendrites (apical, basilar, and oblique). Using these highly averaged values, ASD subjects demonstrated higher average spine densities when compared to age-matched controls. Both layer and dendrite type also had a significant effect on spine density. Pyramidal cells from the superficial layers (II and III) had greater spine densities than those from the deeper layer, and spine densities were greatest on small, oblique dendrites as compared to basilar, but not apical dendrite densities.
Spine densities as a function of distance from the pyramidal cell soma were evaluated exclusively on apical dendrites, since these can often be followed for some distance from the cell body. As expected, densities varied according to distances from the cell body, but there was no interaction between region and distance. Spine densities were again found to be higher in ASD subjects as compared to controls, and this effect did not interact with distance. Within layer II, ASD and control subjects differed from each other in spine density, and these differences were consistent across regions and at varying distances from the cell body (see Fig. 12.2). The only other layer region combination that showed increased spine densities in ASD subjects was layer V of the temporal lobe.


Fig. 12.2
Top: Subjects are presented with bilateral 3 × 3 targets that are either mixed or the same, and then, following a 1,500 msec delay, subjects are shown a single match probe and asked to determine it matches the previously presented target. Bottom: Comparisons of percent correct according to whether the initial target was mixed or the same (redundant). Data for NT adults and callosotomy patient JW (From Holtzman and Gazzaniga 1985, error bars are unavailable). Neurotypical adult performance drops to chance when the targets are mixed and perform close to ceiling when they are the same. Patient JW gets fewer correct overall, but shows almost no cost when the targets are mixed relative to when they are the same. Young ASD subjects and age-matched NTs, show a pattern that is very similar to that demonstrated in callosotomy patient JW
In our sample, density differences between neurotypical and ASD subjects were driven largely by a subgroup of cases. Only seven of the ten ASD cases showed increased averaged spine densities relative to age-matched control cases, and in one instance the magnitude of this difference was very small. Available case histories allowed the assessment of seizure disorders, secondary medical conditions, medications, educational history, level of cognitive functioning, and the severity of specific domains of diagnosis as assessed by a postmortem ADI-R (Lord et al. 1994).
When level of cognitive functioning was considered, each of the four lowest-functioning cases showed increased spine densities relative to their age-matched control. Of the cases identified as either not retarded or mildly retarded, only one had greater spine densities. In two cases, the level of cognitive functioning was unknown, and one showed higher spine densities while the other did not. Many developmental disorders, such as fetal alcohol syndrome (Ferrer and Galofré 1987), severe infant protein-calorie malnutrition (Benítez-Bribiesca et al. 1999), infant brain damage (Dietzmann and von Bossanyi 1994), and Down syndrome (Suetsugu and Mehraein 1980), show spine loss rather than spine increases. These spine reductions are presumed to be associated with connectional loss, abnormal cortical circuits and impaired cognitive abilities (Fiala et al. 2002; Halpain et al. 2005). There are only a few conditions associated with mental retardation where an increase in spine densities has been shown, including fragile X syndrome (Irwin et al. 2001) and hemi-megalencephaly (Takashima et al. 1991). Fragile X and ASD show some notable similarities. Like ASD, fragile X is more common in males than females (Demark et al. 2003), and it shares several cognitive and behavioral traits (Kaufmann et al. 2004). Overall brain size is also larger in individuals with fragile X (Sabaratnam 2000). Estimates of the co-occurrence of fragile X in ASD subjects range from 1.6 to 16 % (Demark et al. 2003), but none of the ASD subjects in our sample carried a comorbid diagnosis of fragile X. Although fragile X and ASD may not share similar causative antecedents, it is interesting that two developmental disorders sharing greater spine densities also share similar behavioral phenotypes and can coexist in the same individual (Demark et al. 2003).
Greater spine densities were also loosely associated with smaller brain sizes, while in our control subjects there was no relationship between spine density and brain size. Although average brain sizes in ASD populations are consistently enlarged in younger individuals between the ages of 1 and 7 years (Courchesne et al. 2003), the adult ASD population is characterized by high variance in brain size, which includes a 20 % rate of megencephaly (Bailey et al. 1993; Filipek et al. 1992; Piven et al. 1995) and average values that do not differ significantly from control groups (Courchesne et al. 1999). As ASD subjects age there are significant reductions in total brain volume (Aylward et al. 2002) and accelerated morphometric changes (Hardan et al. 2004).
Because spines are plastic, many additional factors could contribute to alterations in their density. For example, in both the ASD and control group spine densities decreased with age, although this reduction was not significant. Interestingly, higher spine density tended to be associated with the smallest brains in our ASD group, but not in neurotypical subjects. Spine densities also decreased slightly with increasing PMI time in our control group, but increased slightly in our ASD group and these relationships were nonsignificant.
Spine densities can be reduced by the presence of seizure activity (Multani et al. 1994; Swann et al. 2000), as well as by the neuroleptics used in the treatment of epilepsy (Benes et al. 1985; Garey et al. 1998). In our sample, four of the ten ASD subjects had a history of seizures early in development that were treated with anti-convulsant medications (phenytoin, divalproex sodium, lorazepam, carbamazepine, or phenobarbital). Two members of this group showed greater spine densities relative to their age-matched control, while the other two showed spine densities similar to controls. Although it is unclear whether a relationship exists between spine density, seizure frequency, seizure type, or the medications utilized to maintain seizure control, it is interesting to note that half of the epilepsy cases did not show higher spine densities. Whether medication may have masked an effect in these cases is unknown.
The temporal lobes showed a region-specific elevation in spine density within layer V (Fig. 12.2), a finding that supports a variety of studies implicating the temporal lobes as one of the primary loci of impairment in ASD. Finally, alterations in spine densities within the ASD group did not appear to be associated with a history of epileptic seizure activity, although a weak relationship may exist between high spine densities, the smallest brain sizes, and lower levels of cognitive functioning.
Alterations in spine densities within the deep layers of the temporal lobe suggest that this region may have additional impairments in ASD subjects. Although no single neural structure is associated with ASD, both limbic areas and mesial temporal lobe structures have been identified as having significant neuroanatomical abnormalities in ASD groups (Bauman and Kempter 1985; Bauman 1991, 1996; Bauman and Kemper 1994). These regions are heavily interconnected with adjacent temporal regions (Amaral and Price 1984), and abnormalities in temporal-limbic circuits might be associated with deficits in facial and emotional recognition, as well as associated social-emotional functioning (Critchley et al. 2000; Schultz et al. 2000). These regions also show abnormal activation in auditory studies, theory of mind tasks (Baron-Cohen et al. 1999), and the processing of simple (Gage et al. 2003) and complex speech-related sounds (Just et al. 2004; Boddaert et al. 2004; Gervais et al. 2004). Finally, volumetric and morphometric alterations to temporal lobe structures have also been reported (Boddaert et al. 2004; Bailey et al. 1998; Bigler et al. 2003). In aggregate, these findings point to substantial deficits in temporal lobe circuits that underlie some of the behavioral symptoms that characterize ASD patients.
In sum, ASD is among a small group of developmental disabilities involving mental retardation where there is no loss of dendritic spines. Instead, a substantial subgroup of ASD individuals show increased spine densities relative to age-matched controls. This alteration in cortical circuitry organization is not directly associated with the occurrence of epilepsy but is associated with decreased brain size. In addition, higher spine densities are most often found in the lowest functioning subset of cases examined. The presence of supernumerary spines and increased local white matter volumes, in combination with a lack of functional coherence between distant cortical regions, suggests a model of synapse formation in ASD where experience-dependent strengthening and weakening of neuronal interconnections during the postnatal period is impaired (Mundy and Neal 2001; Courchesne 2004; Frith 2004). Finally, these alterations to the connectional patterns that are established postnatally in the developing human brain may have additional implications for the cognitive strengths and impairments demonstrated in ASD (Happe and Frith 2006) and the success of early behavioral intervention programs (Dawson et al. 2000; Goldstein et al. 2002) that specifically impact the time frame in which the process of synaptic culling is the most active.
Because almost all excitatory neurotransmission in the cortex occurs at synaptic spines, alterations to the number and/or morphology of these spines could disrupt the balance between excitation and inhibition. An increased number of immature spines (much like that of FXS) could cause decreased excitation across networks leading to hypoconnectivity. In fact, an imbalance in the excitation inhibition ratio has been hypothesized to underlie ASD and altered connectivity (LeBlanc and Fagiolini 2011). Animal models for altered neuroligin expression have also been shown to disrupt the balance between excitation and inhibition, which has important implications for synaptic transmission and the motor stereotypies seen in ASD (Hines et al. 2008; Blundell et al. 2010).
Alterations to synaptic density and/or morphology have been found in a number of neurological disorders, including Alzheimer’s disease (AD), schizophrenia, Fragile X syndrome (FXS), and autism spectrum disorders. In AD, for example, researchers note prominent synaptic loss in the cortex of these patients (Baloyannis et al. 2007), which has even been shown to correlate with cognitive severity (DeKosky and Scheff 1990). Furthermore, researchers have also implicated synapse loss within the hippocampus at an early stage of disease progression in individuals with mild AD (Scheff et al. 2007). Similar results have been found in the schizophrenic brain where experimenters have identified a marked decrease in dendritic spines in both the auditory cortex (Sweet et al. 2009) and the prefrontal cortex (Glantz and Lewis 2000). As Penzes et al. (2011) note, Alzheimer’s disease and schizophrenia have disruptions at the synapse in which synaptic loss is consistently found; however FXS and ASD are characterized by a marked increase in dendritic spines. FXS, a disorder highly comorbid with ASD, has been shown to result in an overall increase of dendritic spines as well as a larger proportion of immature spines (Irwin et al. 2001).
Animal models and molecular studies further implicate the synapse as being highly involved in the disruption seen in ASDs. Namely, the neuroligin-neurexin pairing is responsible for the maintenance and function of the synapse with neuroligins presenting at the post-synaptic site and neurexins presenting at the pre-synaptic site. These cell adhesion molecules have been shown to be abnormally expressed in human autistic genetic studies (Jamain et al. 2003; Laumonnier et al. 2004). Furthermore, knockout studies of neuroligins in mice provide a neuroanatomical basis for some of the behavioral phenotypes seen in ASDs with some researchers claiming increased inhibitory synaptic transmission for neuroligin-3 (Tabuchi et al. 2007) and decreased excitatory transmission for neuroligin-1 deletion (Blundell et al. 2010). Other synapse-specific molecules have been implicated in the disorder, such as Shank3 (Durand et al. 2007). These studies provide converging evidence for a disruption at the synapse as being a potentially important factor in the development of ASD, however alterations at these specific markers may be present in only a small subset of the larger ASD population (O’Roak et al. 2012).
12.3 Synaptic Morphology
Although synaptic spines are increased in ASD, the presence of this alteration does not indicate the nature of the pre- and postsynaptic relationship. Spines possess morphological characteristics that have been associated with specific functional roles. For example, variation in shape and length, and the presence or absence of a spine head, have been used to inform the nature of the pre- and postsynaptic relationship at individual dendritic spines. The size of the spine head appears to have a direct relationship with the strength of the synapse such that large heads indicate a strong, consolidated synapse (Yuste and Majewska 2001). Characteristics such as the thickness of the spine neck may underlie calcium compartmentalization allowing spines to independently control biochemical fluxes in and out of the dendrite. Lastly, spine length decreases follow prolonged stimulation and the associated induction of long-term potentiation (Yuste 2011). This previous work informs the stability of the synapse such that large, thick spines are thought to reflect stable synaptic connections, whereas long, thin spines are indicative of immature or malleable connections (Woolfrey et al. 2009; Holtmaat et al. 2005; Irwin et al. 2001).
Importantly, both width and length of the spine control calcium by creating a barrier from the dendrite through which chemical compartmentalization can be independently controlled. These characteristics of the spine can be experience dependent (Nikonenko et al. 2005), are relatable to maturity of the spine (Bourne and Harris 2007; Irwin et al. 2001), and have even been implicated in several neurological disorders (Penzes et al. 2011).
Dendritic spines were evaluated in ASD for length and morphological characteristics, such as thickness and the presence of a spine head. Previous research (Irwin et al. 2001) has subdivided the morphology of dendritic spines into eight different types, including several morphologies that were rarely found in our tissue samples. Most of these spine types are based upon the morphological characteristics of spine thickness and the presence of a synaptic head. In order to encompass most of the variations in these previous systems, we used a three-part classification scheme: length, presence or absence of a spine head, and whether the spine was thick or thin. Using this method we collected morphological data on over 20,000 synaptic spines from three cortical regions and from pyramidal cells located in three cortical layers. Our results revealed that the morphology of apical dendritic spines in ASD subjects differ from neurotypical subjects in two key respects. First, although spines length varied tremendously in both groups, in the ASD cases were longer on average and the distribution of their lengths was positively skewed indicating a greater frequency of long, immature morphologies (see Fig. 12.3). Second, along with the increased lengths, we encountered a greater preponderance of spines with heads, which has also been described as a characteristic of immature spine morphologies (Avino and Hutsler, in prep.).


Fig. 12.3
A comparison of spine lengths as a function of cortical layer for NT subjects and individuals with ASD. ASD subjects show positively skewed distributions and overall greater spine lengths relative to neurotypical subjects. Boxes are the interquartile range and median values, while the whiskers show the 5th and 95th percentiles
Spines were reliably longer in the ASD group and immature spine types were found more frequently in ASD subjects relative to neurotypical controls. Given what is known about the relationship of spine morphology to physiology, these results suggest that, along with the previously reported density increase, there is an overall reduction in the proportion of stable, strengthened spines in the autistic brain. A failure of proper synaptic spine maturation may underlie the deviation from neurotypical spine morphology. Like spine density changes, these disruptions likely reflect abnormal neurodevelopmental maturation and/or abnormal experience-dependent processes. Given the strong link between spine structure and function, both an increase in spine density with concurrent dysmorphology likely contribute to the connectional changes seen in the disorder and point towards the synapse as a major factor in the autistic phenotype.
12.4 Cortical Connectivity and the Subplate
The cortical subplate appears prior to the cortical plate during development and plays a critical role in guiding cortical connectivity. Although many of the neurons within the cortical plate undergo apoptosis after they have served their developmental function, in primates many are retained and appear as a diffuse band of neurons located directly subjacent to cortical layer VI. These neurons maintain connections with the overlying cortex and are integrated into the mature cortical circuitry.
Within 2 months of gestation, subplate neurons are co-generated with cells that will become the Cajal-Retzius cells of layer I, making them amongst the earliest born neurons of the cerebral cortex (Allendoerfer and Shatz 1994). Subplate and Cajal-Retzius cells are also the earliest active neurons in the presumptive cortex with endogenous spontaneous activity that begins around 8 weeks gestation and extends until 18 weeks of gestation. The two cell groups are split into an upper (layer I) and a lower (subplate) compartment by migrating neuroblasts that form the early cortical plate.
Between 13 and 18 weeks gestation the subplate expands in volume due to axonal ingrowth from a variety of fiber types. These include catecholaminergic and serotoninergic afferents originating from the brainstem, cholinergic afferents from the basal forebrain, glutamatergic afferents from the thalamus, and glutamatergic afferents from other cortical locations. Between 19 and 23 weeks of gestation thalamocortical fibers begin to drive activity in the subplate population. It is not until between 23 and 24 weeks of gestation that these afferents will penetrate the overlying cortical plate (Shatz and Luskin 1986). As such, during this time period subplate neurons exhibit mature morphologies and physiological properties, while the layers of the developing cortical plate are still immature (McAllister 1999; Luhmann et al. 2009).
In addition to the suplate’s role in guiding thalamocortical axons, these neurons guide efferent connections from the cerebral cortex. Anterograde DiI labeling has demonstrated in the cat that cortical plate axons project to the thalamus at a relatively late stage in development, well after subplate neurons have made projections into the thalamus. Studies examining the influence of subplate neurons on ‘pioneering’ the corticothalamic pathway from visual cortex have utilized kainic acid injections into the subplate zone followed by H-leucine labeling. In 50 % of the animals that received these lesions, pathways from the visual cortex to subcortical targets including the thalamus were disrupted (McConnell et al. 1994).
Excessive neuronal profiles within the subcortical white matter have been casually reported in neuropathological examinations of ASD cortex for some time (Simms et al. 2009; Bailey et al. 1998; Hutsler et al. 2007). Subplate neuron numbers and densities are difficult to quantitatively assess for two reasons. First, the density of neuronal profiles within the subplate zone is low which creates difficulties for widely accepted stereological sampling methods. Second, the boundary between layer VI of the cerebral cortex and the subplate is often unclear, making it difficult to reliably draw a boundary around the region. Trained raters may disagree upon the boundary placement depending upon their susceptibility to the perceptual pull of excess profiles and their distribution within the white matter. In cases where the boundary is indistinct the problem is exacerbated (Hutsler and Avino 2013). To circumvent these difficulties we quantified the changes is density, as a function of depth, in images centered on the gray-white matter boundary in both ASD and neurotypical subjects. To quantify the transition we fit sigmoid functions to these density profiles (see Fig. 12.4), and found that ASD subjects had indistinct transition zones relative to a NT control group. This finding was apparent in all of the regions examined, which included eulaminate isocortex from frontal, temporal, and parietal locations (Avino and Hutsler 2010). Currently, we are attempting to directly count the number of profiles using computer-generated sigmoid based boundaries in combination with immunuhistochemical labeling for Nuen and several additional antibodies known to morphologically and neurochemical identify this cell population.


Fig. 12.4
Quantification of the transition zone between cortical gray (left-hand side) and white matter (right-hand side) using overlaid sigmoid functions in binary images. Neurotypical subject boundaries (a) were typically more distinct than those found in ASD subjects (b). Scale bar = 100 μm (Reprinted from Avino and Hutsler 2010 with permission from Elsevier)
Excess subplate neurons are not unique to neuropathological descriptions of ASD. Supernumerary profiles have also been found in the dorsolateral prefrontal cortex, parahippocampal gyrus, and the superior temporal gyrus of individuals with schizophrenia (Eastwood and Harrison 2003, 2005). Friedlander and Torres-Reveron (2009) describe inhibitory subplate neurons in the mature brain as ‘gatekeepers’ for cortical signals. An excess of these inhibitory cells in schizophrenia may result in functional disconnectivity between frontal and limbic areas (Kostovic et al. 2011).
Epilepsy, which is commonly comorbid with autism, also shows heterotopic white matter neurons as well as other focal cortical dysplasias (Hildebrandt et al. 2005; Emery et al. 1997). This characteristic in individuals with epilepsy suggests that persistent white matter neurons in autism could be a contributor to comorbid seizure activity. Interestingly, individuals with epilepsy that demonstrate type I focal cortical dysplasias also exhibit blurring of the cortical gray-white matter boundary (Hildebrandt et al. 2005), similar to what has been found in autism (Avino and Hutsler 2010). There are also columnar abnormalities in epilepsy, but these may not be homologous to those found in ASD (Hildebrandt et al. 2005; Casanova et al. 2002).
Subplate neurons serve as an active hub where axons destined for the cerebral cortex accumulate prior to invading the overlying cortical layers (Shatz and Luskin 1986). They are an intermediary through which thalamocortical axons activate the cortex and, as development proceeds, many subplate-layer IV connections are dissolved as thalamic axons begin to make their connections to layer IV directly. In line with this sequence of events, computational modeling studies within the visual system suggest that crude visual maps first develop within the subplate via the retinogeniculate-subplate pathway (Grossberg and Seidman 2006). Activity from the subplate orchestrates the formation of visual maps within layer IV, and then these maps are taught to other cortical layers until most of the subplate is dissolved.
The subplate’s key role in organizing the overlying cortex has also been demonstrated using kainic acid injections into the subplate after thalamic axons have invaded layer IV, but prior to the formation of ocular dominance columns. Kainic acid excitotoxicity disrupts the normal formation of ocular dominance columns and results in the loss of orientation tuning. These injections also disrupt the laminar organization of layer IV, resulting in mistargeted thalamic terminations within layers II and III, and an increase in the activity-dependent expression of BDNF and GAD. These results strongly support the idea that the subplate is a regulator of cortical activity (Kanold 2004).
In the adult, subplate neurons have connections to the overlying cortical plate, and may participate as “amplifiers” or coordinators of afferent signals (Suarez-Sola et al. 2009; Luhmann et al. 2009). Voigt et al. (2001) elaborated a functional role of subplate neurons as one of generating synchronous oscillatory activity that is dependent upon GABAergic subplate neurons. In the newborn rat, calcium-imaging studies have also demonstrated that the subplate mediates synchronous activity (Hanganu et al. 2009).
Subplate neurons in the mature brain have also been described as ‘gatekeepers’ for cortical signals (Friedlander and Torres-Reveron 2009) and regulators of afferent signals that modulate the balance of inhibition and excitation (Kostovic et al. 2011; LeBlanc and Fagiolini 2011).
In sum, experimentation in a variety of animal models has shown that the subplate plays a critical role in several developmental processes, including guiding thalamocortical and corticothalamic axons (Shatz and Luskin 1986), influencing columnar structure (Kanold et al. 2003; Grossberg and Seitz 2003), and generating synchronous activity in the overlying cortical plate (Luhmann et al. 2009; Suarez-Sola et al. 2009; Voigt 1989). The subplate may also play an important role in regulating connectivity in the mature brain and abnormalities of this neuronal compartment may drive disruptions in long-range connections. Because abnormalities of the cortical subplate in autism may provide a microanatomical substrate for the disrupted cortical connectivity theory, there has recently been an increase in interest in this embryonic compartment (McFadden and Minshew 2013).
Several critical questions remain to be answered. First, are the neuronal profiles within the white matter truly subplate neurons, or are they vestiges of cortical neurons that failed to fully migrate? A partial answer to this question is already available, as Golgi staining in the ASD brain demonstrates that many of these profiles have multistellar and horizontally fusiform morphologies, a characteristic of the neurotypical subplate population (Avino and Hutsler, in prep.). Second, what proportion of the subplate population in ASD is GABAergic? Immunohistochemistry for several antigens that are known subplate markers can readily provide this information, and this is an especially important question given previously proposed theories of disconnectivity in the schizophrenic brain that are dependent upon an increased prevalence of inhibitory subplate neurons. Finally, how does this excess of white matter neurons arise during development? Are they a product of failed prenatal apoptosis, or are cells overproduced prior to the formation of the cortical plate and subsequent apoptosis? This question is, of course, difficult to answer in human postmortem material and will require a valid animal model of ASD to properly assess.
Although an early pathology in ASD that may underlie subsequent deviations from neurotypical development is certainly of interest, there is also a practical aspect to further elucidation of the role of the subplate in autism. The subplate region can already be distinguished from the intermediate zone using sonographic techniques (Pugash et al. 2012), and the future possibility of prenatal identification of subplate abnormalities could provide one of the earliest chances to identify children at risk for ASD.
References
Aboitiz F, Scheibel AB, Fisher RS, Zaidel E (1992) Fiber composition of the human corpus-callosum. Brain Res 598:143–153. doi:10.1016/0006-8993(92)90178-C PubMed
Alexander AL, Lee JE, Lazar M, Boudos R, DuBray MB, Oakes TR, Miller JN, Lu J, Jeong EK, McMahon WM, Bigler ED, Lainhart JE (2007) Diffusion tensor imaging of the corpus callosum in autism. NeuroImage 34:61–73. doi:10.1016/j.neuroimage.2006.08.032 PubMed
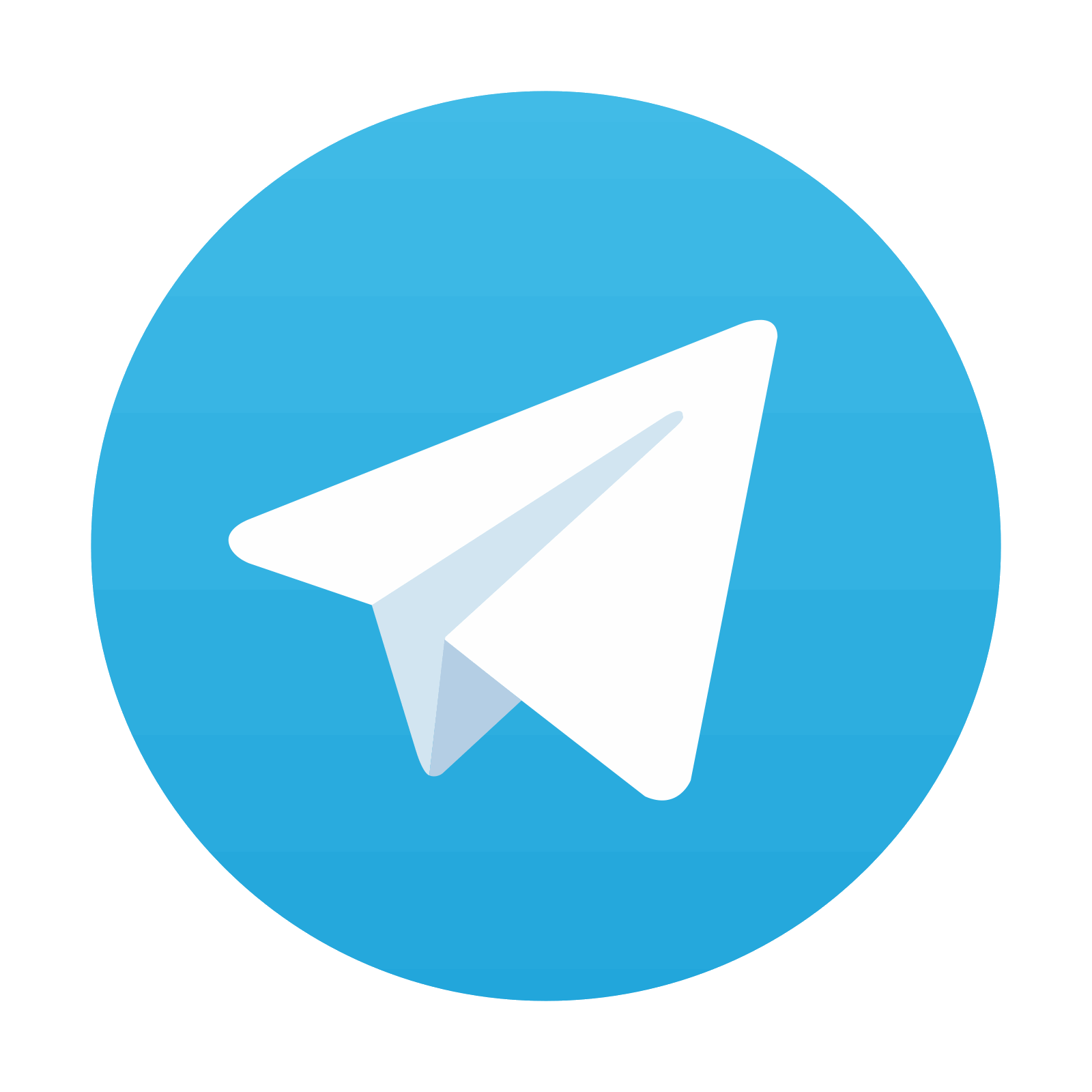
Stay updated, free articles. Join our Telegram channel
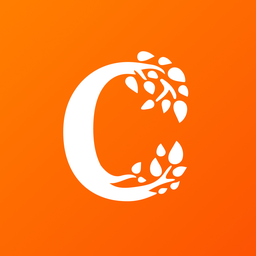
Full access? Get Clinical Tree
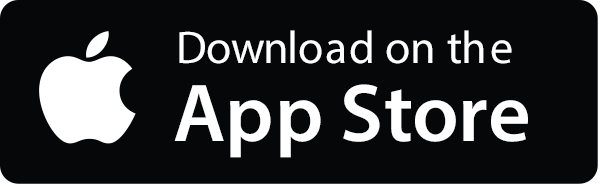
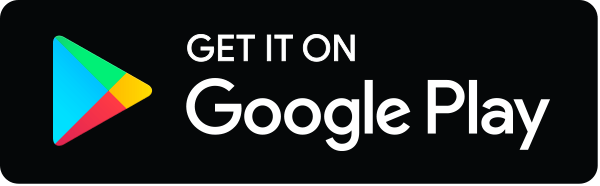