Component
Innate Immune System
Adaptive IS
Cellular
Monocyte, macrophage, granulocyte, NK-cell, γ/δ-cell
T- & B-cell
Humoral
Complement, APP, mannose binding lectin (MBL), neopterin
Antibody
The view that a decline of the immune response might be a pathogenetic factor in AD was discussed earlier when lower levels of pro-inflammatory cytokines, including the mainly monocyte-derived cytokine TNF-α, were described in the CSF of AD patients (Richartz et al. 2005).
The Possible Role of Immune-Driven Tryptophan/Kynurenine Metabolism in AD
The tryptophan/kynurenine metabolism—i.e. the degradation of tryptophan to its partly neuroprotective, partly neurotoxic metabolites such as kynurenic acid (KYNA), 3-hydroxykynurenine (3-HK) and QUIN—is driven by the enzyme indoleamine 2,3 dioxygenase (IDO). Immune mechanisms are key players in this system: IDO is activated by pro-inflammatory cytokines such as interferon-γ or IL-2. An immune activation is associated with an increased degradation of tryptophan and kynurenine. An interesting study investigated whether an imbalance between neurotoxic and neuroprotective kynurenine metabolites could be detected in patients with AD. The study measured serum levels of tryptophan, KYNA, 3-HK, picolinic acid and QUIN in patients with AD and found that the serum levels of 3-HK were markedly higher in AD patients than in the comparison groups (p < 0.0001), while serum levels of the other metabolites of the kynurenine pathway did not significantly differ between groups. 3-HK generates toxic free radicals, leading to neurodegeneration and apoptosis of neurons (Chiarugi et al. 2001; Okuda et al. 1998). In contrast to its downstream metabolites QUIN and picolinic acid, 3-HK can cross the blood–brain barrier via an active transport process. These data therefore indicate an enhanced availability of 3-HK in the brain of AD patients, which may be related to the previously reported higher production of QUIN in AD brains (Schwarz et al. 2013). Accordingly, an immunocytochemical study showed that both 3-HK and IDO—the first, rate-limiting enzyme in tryptophan/kynurenine metabolism—were increased in AD brains compared to controls (Bonda et al. 2010). The increased 3-HK concentration in the serum of AD patients encourages further studies in the search for a peripheral diagnostic biomarker for AD. In this study, the peripheral concentration of QUIN was not elevated, although studies suggest that QUIN—downstream from 3-HK and the product of 3-HK degradation—might also be involved in AD. QUIN facilitates the formation of amyloid plaques and tau phosphorylation, one of the major mechanisms in the generation of neurofibrillary tangles (Rahman et al. 2009), a key factor in AD. Moreover, QUIN is an NMDA receptor antagonist that affects glutamatergic neurotransmission. One study showed increased levels of QUIN in the plasma of AD (Gulaj et al. 2010), but did not find differences in 3-HK concentrations. An explanation could be the stage-dependent increase of QUIN in AD. Increased brain levels of QUIN were also found in AD (Guillemin et al. 2001, 2003), but primarily in early to moderate stages (Guillemin et al. 2007), while no elevated levels of 3-HK or QUIN were found in post-mortem brains of AD, which represent late stages of the disease (Baran et al. 1999).
The balance of the products that result from activated microglia is important for the inflammation process.
To sum up, the results from microglia studies provide clear indications for the important role of neuroinflammation in disease progression in AD. However, some parts of microglia activation might also be beneficial during the course of AD. As explained above, neuroinflammation is a critical event in AD. Consequently, anti-inflammatory therapy has been suggested to be beneficial in delaying the onset or slowing the progression of AD. Cyclooxygenase (COX) is a unique enzyme. First, it exhibits two catalytic activities, bis-oxygenase activity, which catalyses prostaglandin (PG) G2 formation from arachidonic acid, and peroxidase activity, which reduces PG G2 to PG H2. The peroxidase activity also results in the production of free radicals, which are in part utilized by COX itself (Smith et al. 1996). Although NSAIDs may have other effects as well, their primary mechanism of action is generally assumed to be competitive inhibition of COX activity, which reduces the production of inflammatory prostaglandins from membrane-derived arachidonate. COX not only helps mediate production of prostaglandins and other inflammatory factors, but is itself upregulated by pro-inflammatory mediators (Krause and Müller 2010; Aisen and Davis 1997).
In AD, Aβ neurotoxicity may result from several mechanisms, most likely in combination. These mechanisms include oxidative damage, direct cytotoxicity, and induction of destructive inflammatory mechanisms; efforts have been directed at the control of each of these processes (Aisen and Davis 1997), as described below.
Possible Mechanisms of Action of NSAIDs in Alzheimer’s Disease
The treatment of AD with NSAIDs is one of the most promising approaches. If NSAIDs are beneficial in AD, their presumed mechanism of action would be inhibition of COX expressed in the brain, where both COX-1 and COX-2 are expressed. COX-2 plays a unique role in the brain compared to the periphery: only in the brain it is expressed constitutively, whereas elsewhere its expression is activation-dependent. Although in vivo the majority of COX-2 appears to be made in neurons, COX-2 was also seen in rat astrocytes and microglia (Hirst et al. 1999). COX-inhibiting NSAIDs have been shown to reduce microglia activation after infusion of Aβ in rats (Hauss-Wegrzyniak et al. 1999). Neuronal stress, such as ischaemia and excitotoxicity, is associated with strong upregulation of neuronal COX-2 expression, suggesting that COX-2 is involved in neurotoxic mechanisms and may therefore represent a target for drug therapy in the treatment of AD (Planas et al. 1995; Tocco et al. 1997).
Several epidemiological studies provide the background for possible mechanisms of action of NSAIDs in AD. Most studies use COX-2 inhibitors, because neuronal COX-2 is upregulated in response to exposure to Aβ (Pasinetti and Aisen 1998) and focal increases in COX-2 have been shown in the region of amyloid plaques in double transgenic mice carrying genes that encode both mutant APP and mutant presenilin 1 (Matsuoka et al. 2001). Many studies seem to show that COX-2 inhibition confers neuroprotection (Hewett et al. 2000; Willard et al. 2000; Kunz and Oliw 2001; Araki et al. 2001). Some studies have revealed an upregulation of neuronal COX-2 in the brains of patients with AD (Yasojima et al. 1999; Ho et al. 2001), although this has not been a universal finding (Lukiw and Bazan 1997; Chang et al. 1996). Explanations for the variation in COX expression are the short half-life of COX-2 transcripts and individual variability of inflammatory-related processes.
COX-1 is also localized in microglia and is actively involved in brain injury induced by pro-inflammatory stimuli, including Aβ, LPS and interleukins. A study in 20-month-old triple transgenic AD mice showed that their memory function increased after treatment with COX-1 inhibitors and amyloid deposits and tau hyperphosphorylation in the hippocampus decreased (Choi et al. 2013). Trifusal, a platelet anti-aggregant and irreversible COX-1 inhibitor, was able to rescue cognitive deficits by reducing the dense-core amyloid plaque load, associated glial cell activation and pro-inflammatory cytokine levels in a transgenic mouse model (Coma et al. 2010). Unfortunately this process could be clearly demonstrated only in animal experiments.
Another possible principle for the action of NSAIDs comes from the finding that prostaglandin E2 levels are elevated in patients with AD, especially in early stages of the disease (Montine et al. 1999). Therefore NSAIDs that block prostaglandin E2 synthesis might be beneficial. This idea is further strengthened by glial culture studies indicating that prostaglandins, particularly prostaglandin E, alter the production of several inflammation-related molecules, including IL-6, chemokines and APP (Lee et al. 1999; Blom et al. 1997; Fiebich et al. 1997).
In addition to the more traditional inflammatory mechanisms associated with COX, unique functions of COX-mediated damage may also occur in the AD brain. For example, several of the prostanoid products of arachidonate metabolism potentiate glutamate excitotoxicity, and COX-2—overexpressing transgenic mice exhibit increased neuronal susceptibility to excitotoxic insult (Kelley et al. 1999).
Some of the previously mentioned studies of COX in ischaemia also suggest that intraneuronal COX-2 levels may contribute to neuronal death by production of free radicals (Pasinetti 1998). In addition, increased COX-2 levels in AD neurons may directly damage neurons or increase their vulnerability to other detrimental processes occurring in the AD brain (Pasinetti 1998). Thus, the actions of NSAIDs to inhibit COX-mediated production of apoptotic factors by neurons could be one of the mechanisms by which these drugs seem to exert beneficial effects in AD.
Another non-COX-dependent mechanism of NSAIDs is to attenuate inflammatory processes by directly activating the peroxisome proliferator-activated receptor gamma (PPARγ), a receptor and nuclear transcription factor (Jiang et al. 1998; Lehmann et al. 1997; Ricote et al. 1998). PPARγ is a member of the orphan nuclear receptor family. In cells of the monocytic lineage, including microglia, PPARγ acts to suppress the expression of a broad range of pro-inflammatory genes (Jiang et al. 1998; Ricote et al. 1998). Some NSAIDs act as PPARγ agonists, directly binding to it and initiating its transcriptional activity. Activation of PPARγ inhibits the Aβ-stimulated activation of microglia and monocytes and their secretion of pro-inflammatory and neurotoxic products. For example, PPARγ agonists act to inhibit the Aβ-stimulated expression of IL-6 and TNF-alpha (Combs et al. 1999), by microglia and monocytes, and to prevent Aβ-mediated conversion of microglia into an activated phenotype (Krause and Müller 2010; Combs et al. 2000).
A further underlying mechanism of AD pathology is oxidative stress (Ansari and Scheff 2010; Smith et al. 2010), possibly caused by ROS, which are known to be released from activated microglia cells. On the other hand, glia cells can also exhibit antioxidative functions by releasing hemeoxygenase-1 (HO-1) triggered by accumulation of 3-hydroxyanthranilic acid (3-HAA), a downstream product of tryptophan metabolism. The association of neuronal injury in AD and oxidative stress has been demonstrated by over-expression of immunoreactive HO-1 protein in neurons and astrocytes of the cerebral cortex and hippocampus. HO-1 was found to be co-localized to senile plaques, neurofibrillary tangles and corpora amylacea (Schipper et al. 2009). A moderate activation of heme catabolism is widely accepted to be neuroprotective and to contribute to degradation of neurotoxic protein aggregates. Regulatory interactions between HO-1 and COX pathways have also been reported (Alcaraz et al. 2003). However, experimental observations indicate that the extent of HO-1 induction may be critical, because excessive heme degradation may result in toxic levels of carbon monoxide, bilirubin and iron. Pharmacological modulation of HO-1 levels in the brain shows promising results in models of AD and PD (Cuadrado and Rojo 2008).
A further aspect of ROS includes activation of COX-1 and -2, which are blocked by NSAIDs. Daily doses of NSAIDs have been shown to increase circulating levels of antioxidants (Kimura 1997). A study in a rat model of AD suggested that treatment with a COX-2 inhibitor reduces oxidative stress and might therefore be beneficial for the course of AD (Nivsarkar et al. 2008).
Another possible mechanism of effect of NSAIDs has been suggested to be a direct effect on amyloid pathology in the brain by reducing Aβ-42 peptide levels via gamma-secretase activity independently of COX activity (Guardia-Laguarta et al. 2010). Weggen et al. (2001) reported that the NSAIDs ibuprofen, indomethacin and sulindac sulphide preferentially decrease the highly amyloidogenic Aβ-42 peptide produced from a variety of cultured cells by as much as 80 %. However, the lowering effect on Aβ-42 could not be shown for some NSAIDs; instead, an increase in Aβ-42 levels was observed (Kukar et al. 2005). The underlying mechanism of reduction of Aβ-42 by NSAIDs was clarified by Lleo et al. (2004), who demonstrated that Aβ-42—lowering NSAIDs specifically affect the proximity between APP and presenilin 1 and alter the presenelin 1 confirmation in vivo and in vitro, suggesting a novel allosteric mechanism of action.
Anti-inflammatory Treatment Studies in AD
In recent years it has become widely accepted that inflammatory processes are an underlying condition of AD. As a result, a number of clinical trials have investigated different anti-inflammatory treatment regimens. In the following paragraph, we summarize the most import findings in regard first to mainly COX-2 dominant inhibitors and second to COX-1 inhibitors.
COX-1 and COX-2 Inhibitors
A prospective cohort study with 6,989 participants showed that long-term use of NSAIDs protects against AD but not against vascular dementia (in t’ Veld et al. 2001). More recently, Szekely et al. provided very similar findings and concluded that NSAID use reduced the risk of AD more than the risk of vascular dementia but mainly in those individuals with an apolipoprotein E (APO) epsilon 4 allele. This study was performed in over 3,000 subjects aged 65 years and older (Szekely et al. 2008). Not only were selective COX-2 inhibitors shown to be associated with a decreased risk of AD, but a reduced occurrence of AD could also be demonstrated with the mixed COX-1/COX-2 inhibitor aspirin (Anthony et al. 2000). A meta-analysis of 17 epidemiological studies yielded strong, generally consistent statistical evidence that NSAID and steroid use is associated with a reduced risk of AD (McGeer et al. 1996). Vlad et al. investigated 49,349 patients with AD and 196,850 controls and showed that long-term (>5 years) use of NSAIDs is protective against AD. These findings were clearest for ibuprofen, but did not appear for other NSAIDs (Vlad et al. 2008).
Naproxen, which is slightly more selective for COX 1 than COX 2, reduced the risk of developing AD in 117 patients with mild cognitive impairment (MCI) from whom CSF was collected 21–41 months after treatment was terminated. The tau-to-Aβ42 ratio was reduced by more than 40 % in the group treated with naproxen (Breitner et al. 2011). Another NSAID with preferential COX 1 selectivity, indomethacin, also reduced amyloid burden in transgenic mice (Jantzen et al. 2002).
To conclude, at least ten studies show effects of NSAIDs on amyloid burden and inflammation in mice.
In humans, not all studies showed a positive outcome for COX inhibitors. For example, a one-year randomized controlled study failed to find an advantage of the selective COX-2 inhibitor rofecoxib over placebo. The authors argued that their results could indicate that the disease process was too advanced to be modified, because the goal of the study was to slow the progression of dementia in patients with established AD (Reines et al. 2004). No beneficial effect on the occurrence of AD could be demonstrated for another COX-2 inhibitor, celecoxib, in a group aged over 70 years (Martin et al. 2008). Wolfson et al. (2002) looked retrospectively at a case–control population and found no support for a beneficial effect of NSAIDs in AD. However, this negative result may have been caused by an insufficient period of data collection before disease onset.
Passive and Active Anti-Aβ Immunotherapies
In the last few years, most of the pharmaceutical industry’s efforts have been directed towards preventing the production and accumulation of Aβ. The most revolutionary development is the removal of brain β-amyloid via anti-Aβ antibodies. Both passive and active anti-Aβ immunotherapies can clear Aβ deposits from the brain of AD patients. AN1792 was used in AD patients with some indications of clinical efficacy but caused aseptic meningoencephalitis in about 6 % of patients and was therefore abandoned. The next generation of active and passive vaccines has been developed in the past few years and is currently under clinical investigation. The aim of these substances is to clear Aβ deposits from the brain and to stop the progression of AD.
Bapineuzumab, composed of humanized anti-Aβ monoclonal antibodies, is the most advanced substance. It has been tested in two phase II trials and reduced Aβ burden in the brain of AD patients. Some patients, especially apolipoprotein E4 carriers, developed a vasogenic oedema, so that the clinical use of bapineuzumab is limited, especially in higher doses (2 mg/kg). The proposed remedy is to treat AD patients, particularly APOE4 carriers, with lower doses. A large phase III trial is currently being conducted with bapineuzumab which will tell us if passive anti-Aβ immunization is able to reduce progression of the disease (Panza et al. 2011). Of course improvements in vaccine design are needed to improve the safety and efficacy of anti-Aβ immunotherapy. Unfortunately at this point we cannot definitely identify individuals in the preclinical stages of AD, therefore passive immunotherapy is indicated only in patients who have been diagnosed with AD, i.e. who have clinical symptoms. At that point, such individuals have accumulated substantial neuropathology in affected regions of the brain (Cribbs 2010).
Perhaps amyloid PET imaging combined with genetic markers and last but not least clinical symptoms such as MCI will potentially be able to detect individuals with a higher risk for developing AD (Fleisher et al. 2012). The development of valid biomarkers for AD should be the aim of research in the next few years.
Conclusion
Neuroinflammation plays a key role in the pathophysiology of AD. Mechanisms that parallel those encountered in localized peripheral inflammatory responses are identified, along with detailed pathways for how the mechanisms interact. On balance, it is likely that AD neuroinflammation exacerbates AD pathogenesis.
A general treatment principle in neurology and psychiatry, i.e. that as early an intervention as possible leads to the best outcome, seems to be especially true for AD, but appropriate biomarkers such as genetic risk factors or imaging techniques are currently lacking. This problem needs to be solved in the next few years. Until then, we will be unable to start treatment early enough.
Many lines of evidence show that Aβ-induced neuroinflammation is an early event in the neurodegeneration of AD (Craft et al. 2006), because increases in microglia activation were observed in very early stages of AD that disappeared over the course of the disease (Vehmas et al. 2003). The fact that neuroinflammation occurs very early in AD could explain why anti-inflammatory treatment seems to be most efficient as a preventive or early treatment. One reason why an early use of NSAIDs is superior to a late one is that COX expression in the brain decreases over time in AD brains (Yermakova and O’Banion 2001). Another reason is that CSF PG E2 levels in patients with AD are high when their short-term memory scores are just below those of controls, but low in later stages of the disease. These findings further support the hypothesis that inflammatory processes predominate early in Alzheimer’s disease (Combrinck et al. 2006) and therefore require early intervention with anti-inflammatory treatment.
The change in inflammation processes over the course of AD could explain the failure of some prospective clinical trials of selective COX-2 inhibitors, i.e. treatment onset was too late. However, the failure could also be due to drug selection (COX-1 and COX-2 have different effects) and dose and duration of treatment. Drug selection in particular seems essential because some NSAIDs have recently been shown to increase Aβ-42 levels. It also has to be noted that NSAIDs may exert their protective effects via non-COX-inhibitory mechanisms, such as lowering of Aβ levels and activation of the peroxisome proliferator-activated receptor-[gamma] (Aisen 2002). These non-COX-dependent mechanisms might be differentially distributed among different COX inhibitors.
In summary, harmful inflammatory processes seem to dominate AD pathology, but inflammatory subsets also have some beneficial functions. If AD neuroinflammation is approached with realistic expectations and rational drug design, AD patients could significantly benefit from anti-inflammatory treatment, especially with NSAIDs. Another important aspect of treatment could be to utilize not only the efficient treatment properties of NSAIDs in early AD, but also to make use of the neuroprotective aspects of neuroinflammation by applying a combination therapy that maximizes the potential of glial activation. This would include treatment with NSAIDs and drugs that enforce anti-inflammatory and antioxidative properties (e.g. with 3-HAA and HO-1 enhancement).
The most promising therapeutic pathway seems to be the passive and active immunization against Aβ. In the next few years we should be able to detect AD much earlier so that ideally we could start treatment, e.g. by immunization, before clinical symptoms appear.
References
Agrawal A, Agrawal S, Cao JN, Su H, Osann K, Gupta S. Altered innate immune functioning of dendritic cells in elderly humans: a role of phosphoinositide 3-kinase-signaling pathway. J Immunol. 2007;178(11):6912–22.PubMed
Aisen PS. The potential of anti-inflammatory drugs for the treatment of Alzheimer’s disease. Lancet Neurol. 2002;1(5):279–84.PubMed
Aisen PS, Davis KL. The search for disease-modifying treatment for Alzheimer’s disease. Neurology. 1997;48(5 Suppl 6):S35–41.PubMed
Akiyama H, Barger S, Barnum S, Bradt B, Bauer J, Cole GM, et al. Inflammation and Alzheimer’s disease. Neurobiol Aging. 2000;21(3):383–421.PubMedCentralPubMed
Alcaraz MJ, Fernandez P, Guillen MI. Anti-inflammatory actions of the heme oxygenase-1 pathway. Curr Pharm Des. 2003;9(30):2541–51.PubMed
Ansari MA, Scheff SW. Oxidative stress in the progression of Alzheimer disease in the frontal cortex. J Neuropathol Exp Neurol. 2010;69(2):155–67.PubMedCentralPubMed
Anthony JC, Breitner JC, Zandi PP, Meyer MR, Jurasova I, Norton MC, et al. Reduced prevalence of AD in users of NSAIDs and H2 receptor antagonists: the Cache County study. Neurology. 2000;54(11):2066–71.PubMed
Araki E, Forster C, Dubinsky JM, Ross ME, Iadecola C. Cyclooxygenase-2 inhibitor ns-398 protects neuronal cultures from lipopolysaccharide-induced neurotoxicity. Stroke. 2001; 32(10):2370–5.PubMed
Balschun D, Wetzel W, Del Ray A, Pitossi F, Schneider H, Zuschratter W, et al. Interleukin-6: a cytokine to forget. FASEB J. 2004;18(14):1788–90.PubMed
Baran H, Jellinger K, Deecke L. Kynurenine metabolism in Alzheimer’s disease. J Neural Transm. 1999;106(2):165–81.PubMed
Bianchi M, Ferrario P, Clavenna A, Panerai AE. Interleukin-6 affects scopolamine-induced amnesia, but not brain amino acid levels in mice. Neuroreport. 1997;8(7):1775–8.PubMed
Bjugstad KB, Flitter WD, Garland WA, Su GC, Arendash GW. Preventive actions of a synthetic antioxidant in a novel animal model of AIDS dementia. Brain Res. 1998;795(1–2):349–57.PubMed
Block ML, Zecca L, Hong JS. Microglia-mediated neurotoxicity: uncovering the molecular mechanisms. Nat Rev Neurosci. 2007;8(1):57–69.PubMed
Blom MA, van Twillert MG, de Vries SC, Engels F, Finch CE, Veerhuis R, et al. NSAIDS inhibit the IL-1 beta-induced IL-6 release from human post-mortem astrocytes: the involvement of prostaglandin E2. Brain Res. 1997;777(1–2):210–8.PubMed
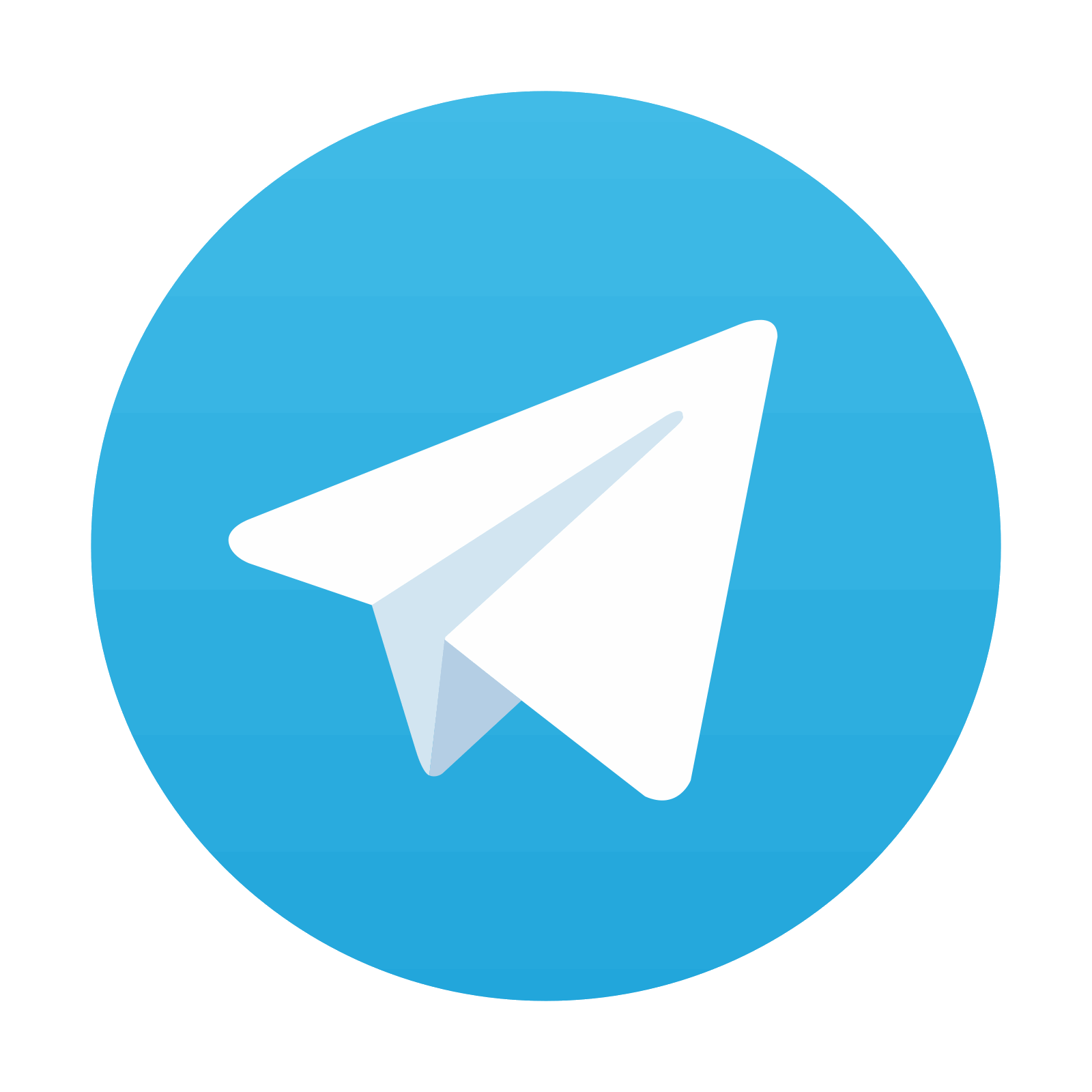
Stay updated, free articles. Join our Telegram channel
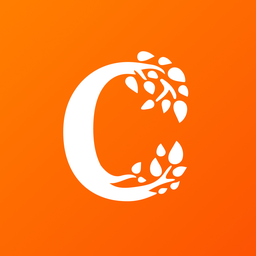
Full access? Get Clinical Tree
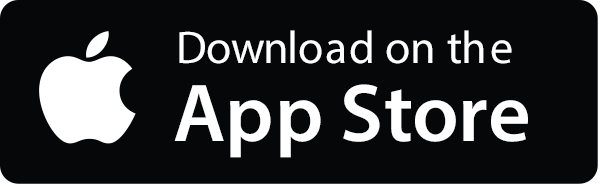
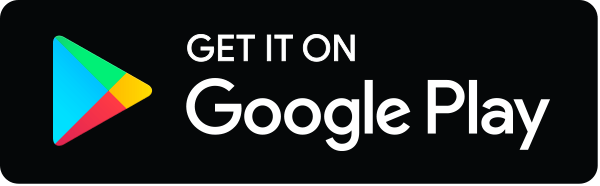