Fig. 4.1
Some of the cortical areas of a squirrel monkey brain that contain columns discussed in this review. Classical columns concerned with either rapidly or slowly adapting afferents are found in the hand (H) representation in somatosensory area 3b (digit territories are numbered). Subdivisions of are 3b (primary somatosensory cortex) for each digit (1–5) or teeth (Te) or tongue (To) are another type of modular organization. Other somatosensory areas include 3a, 1, and 2 of Brodmann (1909). Classical columns have also been well described in primary (V1) and secondary (V2) visual areas, and in other visual areas (see text), which include the third visual area (V3), the dorsomedial visual area (DM), the dorsolateral visual area (DL), the middle temporal visual area (MT), the MT crescent (MTc), the middle superior temporal area (MST) and dorsal and ventral divisions of the fundal area of the superior temporal sulcus (FSTd and FSTv). Inferior temporal cortex (IT) is a higher order visual region. Primary auditory cortex (A1) may have band-like modules for differently processing information from the two ears. Posterior parietal cortex (PPC), primary motor cortex (M1), and dorsal and ventral premotor cortex (PMd and PMv) have larger subdivisions, called domains, related to functionally important complex movements (R-reach, D-defensive movement, G-grasp), as well as a looking domain (not shown) that relates to the frontal eye field (FEF). IT cortex has regions selective for faces or objects. Anatomical studies support that many or all other cortical areas have classical or other types of modular columns, including prefrontal cortex (PFC)
4.2 Minicolumns
Discussions of the features and functions of minicolumns (Buxhoeveden and Casanova 2002) are a special topic of this volume, so they are only mentioned briefly here for the sake of completeness. While definitions may vary somewhat, minicolumns are narrow, vertical arrays of neurons that cross all cortical layers. Neurons are densely connected within the arrays (e.g. Lachica et al. 1992; Yu et al. 2009), and have common response properties, while still differing according to the varied functions and connections of each layer. In some cortical locations, the cell bodies within one minicolumn are somewhat separated from adjacent minicolumns by neuropil (e.g. Buxhoeveden et al. 2001), but in other locations, such separations may not be apparent. The dominance of vertical connections within a minicolumn suggests that they represent a computational unit that is basic to all cortical areas and functions (Lorente de Nó 1949). With the advent of recordings from single cortical neurons with penetrating microelectrodes, it became apparent that vertical arrays of neurons everywhere across the thickness of cortex have some response properties that unites them within a common processing circuit. Local lateral connections also closely tie neurons in one minicolumn with those in adjoining or nearby minicolumns (Krieger et al. 2007). Developmentally, minicolumns appear to reflect the progenitor cells of the ventricular zone that stem clonally and migrate to form the ontogenetic column (Rakic 2007). Once developed, minicolumns vary in width, and are wider in the large human brain compared to similar minicolumns in monkeys (Buxhoeveden et al. 2000). On subsequent pages of this review, we discuss functional evidence for minicolumns in primary motor cortex as parts of larger functional regions called domains.
4.3 Classical Columns
Somewhat differently, Mountcastle (1957) and Powell and Mountcastle (1959) described larger patches of somatosensory cortex that appeared to be columnar in form, extending across the depth of cortex, but wider than minicolumns, varying around 0.5 mm in diameter. These investigators used microelectrode penetrations that coursed tangential to the surface of somatosensory cortex in cats and monkeys to record short sequences of neurons that responded to light touch on the skin or more intense pressure on the skin, and related these responses to presumed differences in activation from superficial skin receptors or deep receptors in muscles and joints. These findings and interpretations led to the widespread search for similar evidence in other cortical areas for neurons that are grouped in patches of cortex extending column-like from surface to white matter, are linked by responding to a common type of input, and alternate with other patches that responded to a different type of input. This interest in modular subdivisions resulted in further evidence for the Mountcastle type of column, but also for other types of columns, all revealed by differences in neuronal activity, anatomical structure, or both. Here, it is useful to distinguish one type of column, the classical column, as columnar patches of cortex that respond to one category of input, while insulated from each other by patches of cortex that respond to a different category of input. The patches might vary in size and shape, but they should involve neurons across cortical layers, and repeat within a cortical area many times. Typically, two or more types of such a column would represent small parts of the same receptor surface, but respond differently due to activation by separate classes of peripheral receptors, or by afferents from functionally different classes of computational outcomes in central nervous system processing.
While some investigators continued to characterize neurons in primary somatosensory cortex of cats and monkeys as responsive to deep or superficial receptors on the body, evidence from other studies suggest that somatosensory cortex is activated throughout by superficial cutaneous receptor inputs, and that alternating columns related to either deep or superficial receptors do not exist in primary somatosensory cortex (e.g. Dykes et al. 1980; Favorov and Diamond 1990; Pons et al. 1987; Sur et al. 1981; Krubitzer et al. 2004). The reason for this discrepancy is unclear, but one possibility is that varying anesthetic levels raised or lowered stimulus threshold levels, leading to cutaneous activation that required more intense stimulation, which was classified as related to deep receptors. It now appears that deep receptor inputs from muscle spindles and joint receptors are relayed over a separate pathway to the ventroposterior superior nucleus of the thalamus to areas 3a and 2 of somatosensory cortex of primates (Cusick et al. 1985; Kaas 2011). Alternations of deep receptor and cutaneous receptor activation territories have been reported for both areas 3a and 2 (McKenna et al. 1982). However, most neurons in both areas are likely activated by proprioceptive inputs from the thalamus and tactile inputs from area 3b and 1 (Pons et al. 1985; Krubitzer et al. 2004).
Nevertheless, another type of modular organization has been proposed for primary somatosensory cortex, area 3b, of primates. Inputs from the skin involve two major types of afferents, the slowly adapting (SA) type I and the rapidly adapting (RA) type I afferents, related to Merkel’s disk and Meissner corpuscle receptors, respectively. These two types of afferents activate separate groups of neurons in the dorsal column nuclei and the ventroposterior nucleus (Kaas 2011), although the modular organization of these groups at each level is yet unknown. However, in area 3b of monkeys, separate groups of cortical layers and neurons appear to be activated by either SA or RA inputs, thus involving columns of neurons that process either SA or RA information (Sur et al. 1981, 1984; Friedman et al. 2004). As these alternating types of columns (Fig. 4.2) represent overlapping distributions of SA and RA receptors in the skin, and are differently responsive to tactile stimulation, they can be regarded as columns of the classical type. Whether they exist or not in primary somatosensory cortex of all primates, or any nonprimate mammals, is yet uncertain.


Fig. 4.2
Evidence for classical columns in somatosensory cortex of owl monkeys. (a). A dorsolateral view of the owl monkey brain with the primary somatosensory area, S1 proper, which contains areas 3b and 1, outlined. The region within the square is shown at higher magnification below. (b). The somatotopic organization of areas 3b and 1 in somatosensory cortex of owl monkeys. In the hand representation of area 3b, the digits are represented from the thumb (D1) to the little finger (D5) in a lateral to medial sequence, and again in area 1. (c). An enlarged view of the representation of digit 4 (D4) in area 3b shows the separate territories that are responsive to either rapidly adapting (RA) or slowly adapting (SA) cutaneous afferents subserving digit 4. The dots indicate the locations of electrode penetrations that sampled neurons in the cortex. (d). The glabrous surface of the monkey hand with the locations of receptive fields from SA or RA neurons recorded within the cortical representation of D4 in area 3b. Note that the digit is represented twice, once in SA territory and once in RA territory. Based on the results of Sur et al. 1981, 1984
The barrels of somatosensory cortex in some rodents may be considered as another example of classical columns. The ‘barrel’ is an unusual term given to one unit of a set of repeated structures, approximately shaped as such, in the primary somatosensory cortex of rats, mice, and a few other rodents (Woolsey and Van der Loos 1970) and some marsupials (Waite et al. 1998). Each barrel can be identified by a dense packing of neurons and higher levels of the metabolic enzyme, cytochrome oxidase (CO) (Fig. 4.3), and represents or is isomorphic with a single whisker on the face, such that neurons in a barrel are driven mainly by the movement of its associated whisker, although adjacent whisker movements may reduce the overall response (see Ebner and Kaas 2015). By themselves, barrels would not constitute a classical column as the same types of input activate each barrel, they simply derive from different whiskers. However, the barrels are separated from each other by narrow septal regions, which get different inputs from the thalamus, have different cortical connections, and contain neurons that have different response properties. For example, neurons in barrels are best activated by touch on a single whisker, while neurons in septa are responsive to many whiskers. Thus, the whisker region of the face is represented once within the barrels and again within the septal regions around the barrels. While the barrels and septal regions are of different proportions, neuron packing densities, and shapes, they do constitute parts of separate, but interacting, processing streams for information from the large sensory whiskers of the face (Alloway 2008). However, barrels vary in size and number of neurons according to the whisker they represent (Meyer et al. 2013). Thus, they do not form an array of identical modules, but are variable and computationally specialized for the different functions of whiskers according to position in the face. In addition to the well-known barrels, other parts of the body are represented in isolated patches of cortex in primary somatosensory cortex of rats (Dawson and Killackey 1987). Thus, barrel-like structures are histologically apparent for other long sensory whiskers on the head and other parts of the body, and smaller structures can be seen for the smaller whiskers of the buccal pad. Narrow, cell poor septa separate these “barrels”, and other groups of neurons that represent digits and glabrous pads of the forepaw and hindpaw. Whether these narrow septal regions constitute a second set of processing modules is uncertain, and there is no evidence that the cortex representing the fur bearing skin of the body is subdivided by septa as well.


Fig. 4.3
A surface view of a small part of the barrel field of primary somatosensory cortex, S1, of a rat. The brain section, cut tangential to the brain surface, has been processed for the metabolic enzyme cytochrome oxidase (CO). This preparation reveals the CO-dense “barrels”, one for each of the mystacial vibrissa of the contralateral face. The barrels are separated by CO-light septa. As the septa and barrels have neurons with different response characteristics, they can be considered to represent two types of classical columns. Scale bar is 250 um
It is important to recognize that narrow, cell-poor septal regions separating the representations of distant body parts are commonly observed in primary somatosensory cortex of mammals, and sometimes in other cortical areas as well (Kaas and Catania 2002). Most notably, the unusual star nosed mole, with 11 fleshy appendages extending from the face around the nostril, has the representation of each appendage or ray separated from those for adjacent rays in primary somatosensory cortex by a narrow septum. In a similar manner, the re-representation of rays in the second somatosensory area S2 is divided by septa as well (Catania and Kaas 1995). Even the digits of the forepaw are partly separated by septa in S2. These narrow septa have few neurons, but are favored by corpus callosum connections. Thus, septal regions could be considered as a second set of modules. Finally, similarly narrow septa separate the representation of digits in the primary somatosensory cortex of monkeys (Jain et al. 1998; Qi et al. 2011; Liao et al. 2013), and other patches of cortex for the teeth and for the tongue are separated by cell-poor regions as well (Kaas et al. 2006; Cerkevich et al. 2013).
A third type of modular organization exists in the large amount of cortex devoted to the representation of the bill of the duck-billed platypus (Krubitzer 1995). The ancestors of the aquatic platypus evolved a new receptor on the bill, one extremely sensitive to weak electric currents. Thus, electroreception became one of the sensory inputs from the skin of the bill, allowing them to detect the weak currents generated by muscle contractions of their underwater prey. As there wasn’t a system already present for electroreception when electroreceptors evolved, this new modality was mediated by the somatosensory system, although in a segregated pathway. Thus, information from the electroreceptors was segregated from tactile inputs in the trigeminal complex, the ventroposterior nucleus, and in somatosensory cortex (Krubitzer 1995). In the huge representation of the bill, occupying as much as one third of neocortex, interdigitated bands of S1 respond to touch and electroreception. Although the shapes of the modules are band-like, rather than columnar, they conform to the definition of classical columns.
Visual cortex also has versions of classical columns. As a well known example, the subdivisions of layer 3 in primary visual cortex of primates into small cytochrome oxidase (CO)- dense patches surrounded by CO-light cortex is well known (Casagrande and Kaas 1994). These patches are found in all primates (Preuss and Kaas 1996), but not in the close relations of primates (tree shrews, rodents, and lagomorphs). Surprisingly, similar structures evolved independently in cats and ferrets (Wong-Riley 1979; Murphy et al. 1995; White et al. 1999). In primates, the dark CO-dense puffs (Carroll and Wong-riley 1984) or blobs (Horton and Hubel 1981) in layer 3 are a reflection of dense, focused terminations of inputs from the koniocellular layers of the lateral geniculate nucleus, the so-called third visual pathway that, in diurnal primates, is dominated by chromatic signals from S (blue) cones (Shostak et al. 2002). Hence, neurons in blob columns are especially involved in color vision, while neurons in the interblob regions are more involved in form vision. A problem for this general interpretation is that nocturnal owl monkeys and prosimian galagos, without blue cones or color vision, have blobs. Thus, it may be more correct to conclude that blobs are part of a pathway mediating contrast, whether color or brightness (Allman and Zucker 1990). This may also be the case for the independently evolved “W-cell” pathway to blobs in cats and ferrets (LeVay and Gilbert 1976), which lack color vision as well (Van Hooser 2007).
In primates, the layer 3 blobs (Fig. 4.3) are not only CO-dense, but can be distinguished as myelin-dense patches in the otherwise even distribution of myelinated fibers in layer 3 (Rockoff et al. 2014). Additionally, blobs correspond to patches of dense labeling of the neurotransmitter transporter VGLUT2, which is found in the terminations of lateral geniculate axons. Inputs from the magnocellular and parvocellular geniculate layers form a continuous distribution in layer 4, and inputs from the koniocellular geniculate layers form the blob pattern in layer 3 (Wong and Kaas 2010; Balaram et al. 2011; Garcia-Marin et al. 2013; Bryant et al. 2012). Because of their vertical connections within cortex, the blob columns and the surrounding interblob columns involve all cortical layers. As functional components of partly segregated systems, blobs and interblobs have differences in inputs, connections within V1, callosal connections, and connections with extrastriate cortex (Casagrande and Kaas 1994). Thus, the blobs and interblobs of V1 can be considered as alternating classes of classical columns (Fig. 4.4).


Fig. 4.4
An example of a pattern of cortical connections that provides evidence for a modular organization of primary visual cortex, or V1. The injection of a retrograde and anterograde neural tracer, cholera toxin subunit B, CTB, into dorsal V3 (solid dark oval) of an owl monkey labeled a regular array of patches of cortex in primary visual cortex, V1, and a less uniform array in the second visual area, V2. Cortex was flattened, cut, and processed parallel to the pial layer to provide a “surface” view of the three cortical visual areas and their regions of label. The patches in V1 likely correspond to the cytochrome oxidase (CO) blobs of V1, while the patches in V2 likely mark the CO-dark thin stripes. The selective labeling of blobs in V1 suggests that V3 has a modular organization, with one set of modules connecting to the V1 blobs. Similar results have been published by Krubitzer and Kaas 1993; Beck and Kaas 1998; and Angelucci et al. 2002
Classical columns are also found in other visual areas of primates. For example, the second visual area, V2, of most primates is characterized by a repeating series of CO-dense thin stripes and a similar series of CO-dense thick stripes that are separated by CO-pale interstripes (Fig. 4.5) (Casagrande and Kaas 1994). Thus, there are twice as many CO-pale interstripes as CO-dense thin and thick stripes. Overall, there are about 30 sets of stripes in V2 of macaque monkeys (Olavarria and Van Essen 1997) and likely more in V2 of humans. Early studies focused on deriving the connections and functional properties of thin, thick, and pale stripes, assuming three types of modules (DeYoe and Van Essen 1985; Livingstone and Hubel 1984, 1987; Shipp and Zeki 1985; Sincich and Horton 2002). More recent evidence indicates that the interstripes are of two types, differing in connections from V1 (Federer et al. 2013), and thereby having different functional properties (Xu et al. 2004). As the visual hemifield would need to be separately represented in each of the four sets of stripes, V2 contains four fragmented visual representations (Roe and T’so 1995), with a global visuotopic organization of the lower visual quadrant through the upper visual quadrant, arranged mediolaterally across the cortical surface. Thus, a set of four adjacent stripes – a CO-dense thick stripe, a CO-dense thin stripe, and two CO-pale interstripes – would combine to form a single hypercolumn of the sort described by Hubel and Wiesel (1972, 1977). Optical imaging in V2 of owl monkeys (Xu et al. 2004) revealed that the medial interstripes in each set are highly selective for stimulus orientation, while the lateral interstripes in each set are not. The CO-dense thick stripes, also sensitive to stimulus orientation, receive inputs from layer 3C of the magnocellular pathway in V1, and project to the orientation sensitive middle temporal area (MT), while the CO-dense thin stripes are sensitive to luminance changes (Kaskan et al. 2009) and to color (Hubel and Livingstone 1987) in those primates with color vision. Although stripe shaped, and crossing the width of V2, the four functionally and anatomically different stripes of V2 qualify as classical columns

.

Fig. 4.5
The blob and interblob modules of V1 in primates differ in functional properties, thalamic inputs, and (as shown here) projections to band-like modular subdivisions of V2. The projections from V1 blobs carry information abut stimulus contrast and color to V2 thin stripes, while projections from V1 interblob regions carry information about stimulus orientation from magnocellular and predominantly parvocellular geniculate inputs to the thick stripes and interstripes, respectively. See text for details
Less is known about the understudied area V3 (Kaas and Lyon 2001). However, optical imaging experiments in owl monkeys, where much of dorsal V3 is exposed on the brain surface, have revealed the existence of a pattern of orientation selective stripes across V3 that are wider than those in V2 (Xu et al. 2004; Kaskan et al. 2009). Parts of these orientation stripes were sensitive to luminance change or sensitive to binocular disparity. In addition, a band-like or patchy pattern of CO-dense cortex has been observed in V3. Connection patterns also suggest the existence of modular subregions with inputs from blob or interblob divisions of V1 (Fig. 4.4). Thus, functionally distinct classical columns appear to exist in V3.
The middle temporal visual area, MT (Fig. 4.1), is a widely recognized visual area of primates that is easily distinguished by its systematic representation of the contralateral visual field and dense myelination (Allman and Kaas 1971). Optical imaging experiments in owl monkeys and prosimian galagos, where MT is exposed on the brain surface and available for imaging, reveal that MT has orientation sensitive neurons throughout, and these are grouped according to their preferred stimulus orientation (Malonek et al. 1994; Xu et al. 2004, 2006). In addition, patches of neurons that are selective for orientation are subdivided into smaller regions that are selective for motion, either in one direction orthogonal to the orientation preference or the opposite direction orthogonal to the orientation preference (Kaskan et al. 2010). This evidence suggests that MT has two types of alternating modules, where orientation selective neurons preferring movement in one direction are grouped together and adjacent to modules preferring the opposite direction of movement. Other evidence is limited (Born and Bradley 2005), but the connection pattern of MT with the adjoining dorsal division of FST is patchy (Kaas and Morel 1993), suggesting a pattern of alternating patches of cortex, with one set of patches projecting to FST and the other not. Similarly, the belt-like MTc area surrounding much of MT is characterized by a series of CO-dense patches of cortex separated by CO-light cortex, and also contains patchy connections with the ventral division of FST (Kaas and Morel 1993). Thus, MTc appears to be subdivided by alternating patches and interpatches of cortex that are likely functionally distinct, and can be classified as classical columns.
Another well studied visual area, the dorsolateral (DL) visual area (Allman and Kaas 1974) or V4 (Zeki 1973) is located between V3 and MTc (Fig. 4.1). DL/V4 represents the contralateral lower to upper visual hemifield in a dorsoventral cortical sequence (Allman and Kaas 1974; Gattas et al. 1988), and receives a matching visuotopic pattern of input from V2 (Stepniewska et al. 2005a). Recent optical imaging results from macaques provide evidence that modular regions in V4 alternate to represent either stimulus color or orientation (Tanigawa et al. 2010) and color regions are organized to represent dimensions of hue, lightness, and saturation (Li et al. 2014). The color and orientation modules appear to fulfill the requirements of classical columns, while the complex arrangement of hue, lightness, and saturation representations deserves further study. In addition, the possible arrangement of separate modules for faces or objects in IT of temporal cortex (Fig. 4.1) in macaques suggests that this cortex may have classical columns as well (Tsao et al. 2003; Moeller et al. 2008; Tsao et al. 2008).
In conclusion, as more is learned about the internal organizations of cortical areas, evidence for classical columns has accumulated. Most is now known about the well studied visual areas, and very little about auditory areas, although bands in primary auditory cortex of cats that are excited by both ears (E-E bands) or excited by one and inhibited by the other (E-I bands) have been described (Imig and Adrian 1977; Middlebrooks et al. 1980). The patchy cortical connections from single locations in frontal cortex (Goldman and Nauta 1977) and elsewhere, as well as the modular structure of some cortical regions (Manger et al. 1998), suggest that many more examples of classical columns will emerge, and that they are present in many cortical areas.
4.4 Columns That Spatially Represent a Stimulus Dimension – Unbounded Columns
A number of visual areas, V1, V2, V3, MT, and possibly DM, have regions of cortex that systematically represent stimulus orientation for a given region of visual space. Similar adjoining arrays represent adjoining visual spaces. The hypercolumn of Hubel and Wiesel (1977) is a well-known attempt to portray the continuous change of orientation preference that is revealed by electrode penetrations that cross the vertical arrays of neurons in cortex. In this well known method, the “boundaries” between vertical arrays of neurons with slightly different orientation preferences reveal progressive changes in preferred orientation until the full set of 180° of preferred orientation occurs for stimuli in a given place in the visual field. Other such arrays represent stimulus orientation for other spaces in the visual field, resulting in a large number of hypercolumns for V1 of larger primates. Because the changes in orientation preference across cortex appear to be largely gradual and continuous, the illustrated borders of the so-called orientation columns are arbitrary, and they are usually portrayed as about the width of a minicolumn. If they are to be considered columns, they need to be defined in a way that makes their borders less subjective. As it is, the portrayed orientation columns do not conform to the definition of a classical column, because they are not separated by columns with another class of inputs. However, a full set of orientation columns has at least some definable boundaries. One full set of orientation selective regions constitutes a type of column known as a pinwheel, where orientation preferences continually change through 180° around a point or singularity in cortex (Blasdel 1992). Thus, orientation preferences change sharply across that critical point. Other sorts of discontinuities occur in orientation maps, and these help define the borders of orientation “hypercolumns”, but without some type of separating column with different inputs, orientation columns may not exist as classical columns, but only as a continuously mapped response feature of cortical neurons, much like visual space or auditory tones would be represented in areas without classical columns.
Interestingly, while orientation hypercolumns and the systematic representation of orientation is a feature of V1 and other cortical areas in all primates, and in V1 of tree shrews (Bosking et al. 1997), which are a close relative of primates, they do not exist in V1 of rodents (Van Hooser et al. 2005), which are only slightly more distant relatives of primates (Kaas 2012a, b). However, orientation selective columns have been independently evolved (see Kaschube et al. 2010) in V1 and V2 of carnivores, including cats (Bonhoeffer and Grinvald 1991; Crair et al. 1997) and ferrets (Rao et al. 1997; White et al. 2001).
4.5 Ocular Dominance Columns and Other Modules Representing Separated Parts of Sensory Surfaces
Sensory surfaces may be discontinuous or have disruptions that can be reflected in cortical representations. For example, most mammals relay separate representations of the binocular visual hemifield for each eye from the dorsal lateral geniculate nucleus (Kaas et al. 1972) to a visuotopic representation in primary visual cortex, but they do this in different ways. In some mammals, the relay of the segregated right or left eye inputs to primary visual cortex is often merged in layer 4 of V1, while being distinct at the single neuron level, or at the sublaminar level as in tree shrews (Fitzpatrick 1996). Alternatively, the inputs from one eye or the other are often segregated in a patch and surround, or a band-like pattern in layer 4 of V1 (Fig. 4.6

), and each input may be the dominant driving source of activity in other layers, giving rise to the concept of ocular dominance columns (Hubel and Wiesel 1968). Present evidence suggests that some form of patchy to band-like segregation of neuron groups by ocular dominance is common to many mammals, including carnivores (Löwel and Singer 1987; Anderson et al. 1988; Issa et al. 1999), but especially in primates. However, the form of ocular dominance columns is highly variable, even within V1, and sometimes across individuals of a species (Horton and Hocking 1996; Horton and Adams 2005). Very pronounced ocular dominance columns are revealed by the sharp segregation of geniculate inputs related to one eye or the other in layer 4 of V1 in Old World monkeys, apes and humans (Horton and Hedle-Whyte 1984; see Florence and Kaas 1992, for review). For most of V1, these segregated inputs take the form of meandering bands for right or left eye inputs that merge and part in various ways. In this large part of V1, the inputs from each eye are equal, but in the part of V1 devoted to peripheral vision, the input from the ipsilateral eye is reduced and this results in a dot-like pattern of ipsilateral eye dominance surrounded by a continuous field of contralateral eye dominance (Tanaka 1991). Of course, these segregated inputs from each eye selectively activate the neurons in bands of cells immediately above and below them, so the ocular dominance bands extend from white matter to cortical surface. This is clearly revealed by similar patterns of expression of activity-dependent genes when input from one eye is blocked with tetrodotoxin (TTX; Takahata et al. 2009). The alteration in gene expression also reveals a previously undetected feature of ocular dominance columns. In layer 4, neurons at the border of an activated column adjacent to a deactivated column form a “border strip” where neurons are activated at a higher level than neurons elsewhere in the activated column. This is because they receive most or all of their activating input from the intact eye, while roughly half of their inhibitory surround inputs are from the deactivated eye. For most neurons along the border of a column, inhibition comes from the adjoining neurons of the activated and the deactivated column, while neurons in central locations in a column receive most of their inhibition from within the column itself (Takahata et al. 2009).

Fig. 4.6
Ocular dominance territories are revealed in V1 of a baboon with a longstanding visual deficit in one eye, via immunolabeling for parvalbumin and vesicular glutamate transporter 2 (VGLUT2). Both markers are robustly expressed in the terminals of LGN neurons that synapse in layer 4 of V1. Thus, the dark parvalbumin- and VGLUT2-positive patches in layer 4 correspond to active LGN terminations from the intact eye, while the light patches correspond to inactive terminations from the deprived eye. Scale bar is 1 mm
In New World monkeys and prosimian primates, the expression of ocular dominance columns is highly variable. Some of the larger New World monkeys, such as cebus and spider monkeys, have ocular dominance columns that are just as pronounced, and of the same band-like form, as in Old World monkeys (see Florence and Kaas 1992 for review). In contrast, New World squirrel monkeys have an individually variable pattern, ranging from ocular dominance patches of various sizes to a salt and pepper representation with no obvious dominance across a group of neurons (Livingstone 1996; Adams and Horton 2003). Some marmosets may have highly overlapping distributions of inputs from the two eyes in V1 as adults, but partly separated inputs early in development or after rearing with monocular deprivation, while other mature marmosets have patent ocular dominance columns that may not be apparent in the distributions of lateral geniculate inputs to layer 4, but may be revealed by optical imaging of V1 after blocking vision in one eye (Roe et al. 2005). Nocturnal owl monkeys have a variable and very weak segregation of eye specific geniculate inputs to V1 (Kaas et al. 1976), but these otherwise cryptic ocular dominance patches can be revealed in V1 of owl monkeys by optical imaging (Kaskan et al. 2007) or activity-dependent gene expression after blocking vision in one eye (Takahata et al. 2014). In Fig. 4.7, the normally cryptic ocular dominance columns in an owl monkey are revealed by visually depriving one eye for a short period (1–3 h) and labeling for c-fos, an immediate early gene that is up- or downregulated by V1 neurons in response to visual activity. Thus, the dark patches correspond to ocular dominance columns for the deprived ipsilateral eye, which contain little or no c-fos expression, while the light patches correspond to ocular dominance columns for the normal contralateral eye, which contain elevated levels of c-fos expression. The monocular segment of V1, which gets activated only by the contralateral eye, shows normal levels of c-fos expression, while the monocular projection from the optic disc of the retina, which gets input only from the ipsilateral eye, is downregulated as well. The expression of activity-dependent genes reveals that owl monkeys, as for macaques, have border-strip neurons with higher activity along the layer 4 margins of activated columns with deactivated columns. In cats, the patchy ocular dominance columns extend into V2, and this may also occur in owl monkeys (Takahata et al. 2014). In cats, callosal connections preferentially originate in ocular dominance columns for the ipsilateral eye, except for the border zone between V1 and V2, where they originate from ocular dominance columns from the contralateral eye instead (Olavarria 2001).


Fig. 4.7
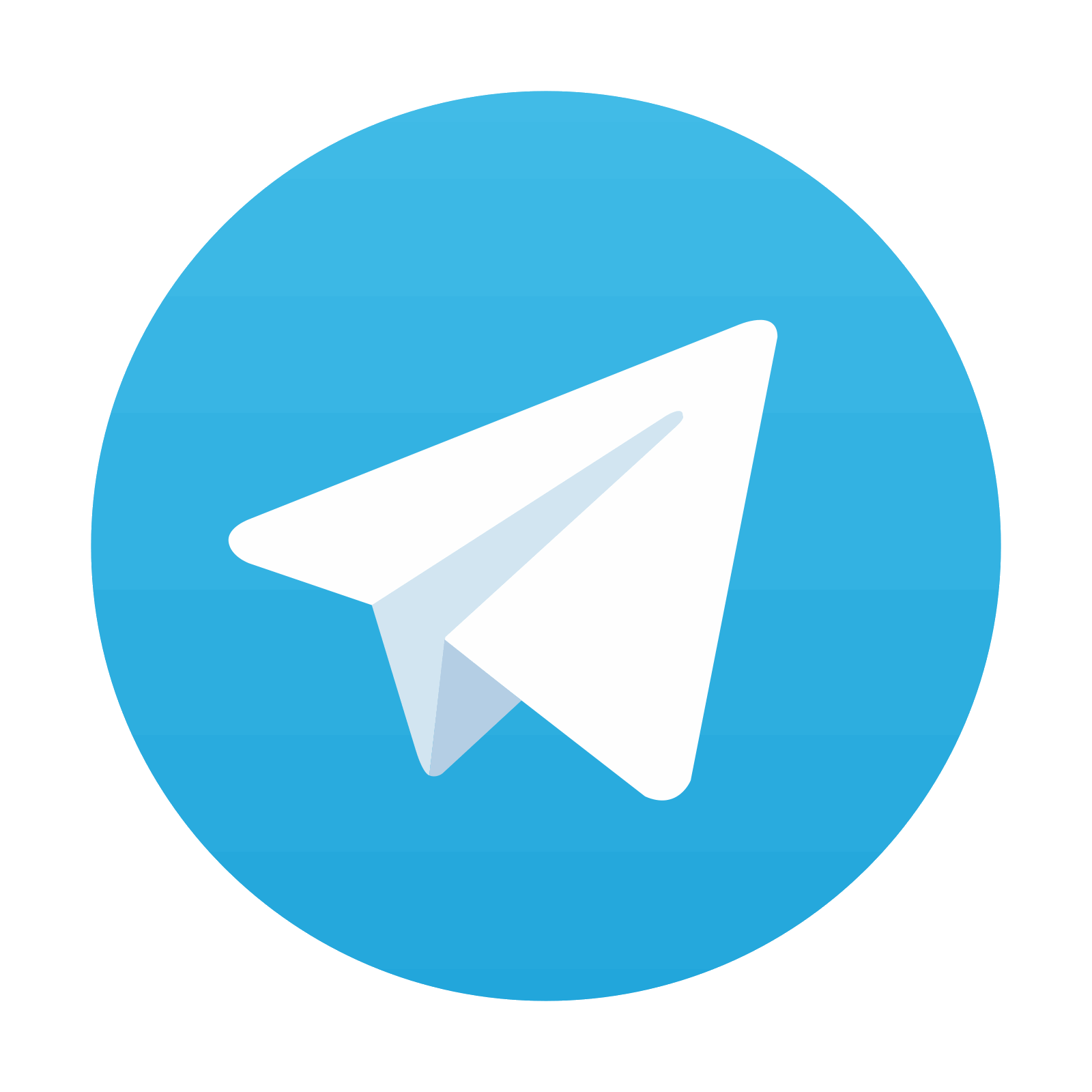
A surface view of part of V1 in an owl monkey reveals the ocular dominance columns related to the ipsilateral eye (dark patches) and the contralateral eye (light patches), after visually depriving the ipsilateral eye for 1–3 h. Note the dominance of the contralateral eye in terms of cortical territory, and the wedge of continuous contralateral eye territory corresponding to the monocular visual field (MF) of the contralateral eye. Scale bar is 1 mm
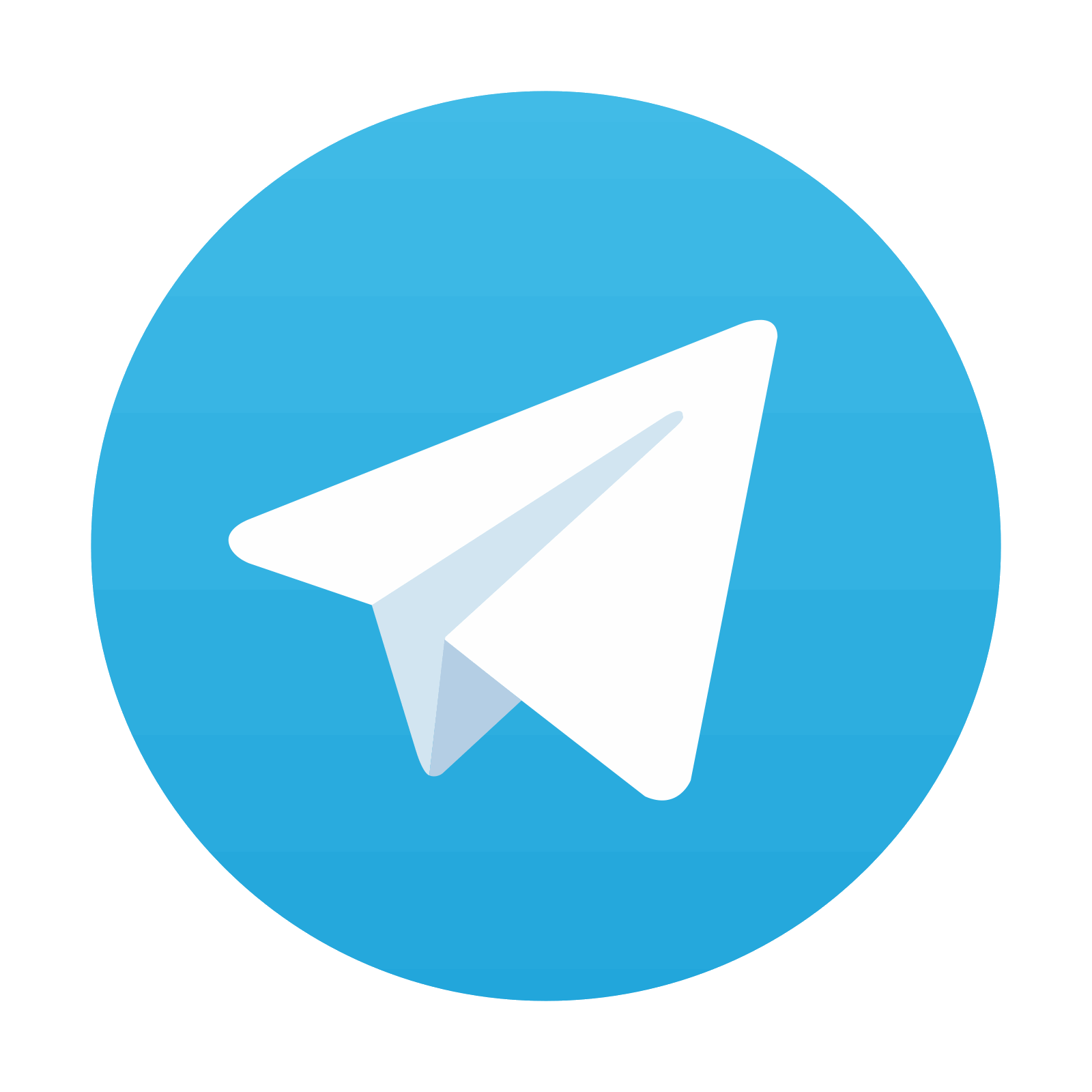
Stay updated, free articles. Join our Telegram channel
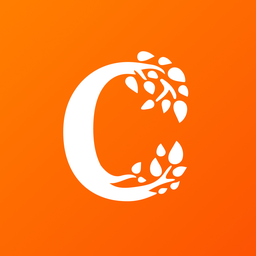
Full access? Get Clinical Tree
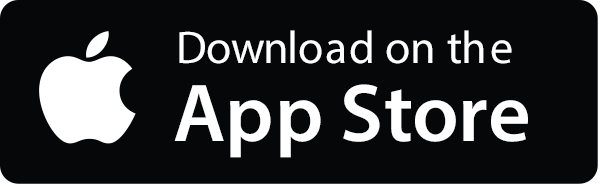
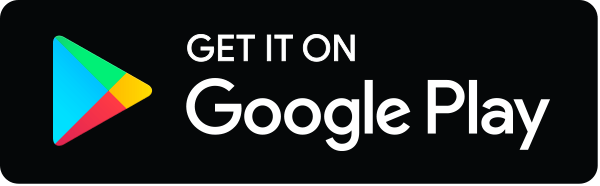
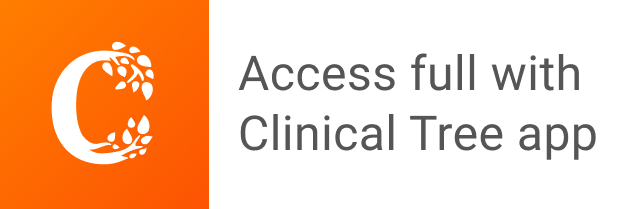