Surgery remains one of the oldest and still most important forms of treatment for patients with glioma. The advantages of surgical resection for glioma must be balanced with the potential of operative morbidity to surrounding eloquent brain. To that end, advances in functional brain mapping allow for safer operations with more aggressive surgical resections. A brief history of motor mapping as well as its present day use in aiding resection of eloquent gliomas is discussed.
One of the oldest and still most important forms of treatment available for patients with glioma is surgery. Even in the contemporary milieu of multimodal regimens, including radiotherapy and chemotherapy, glioma resection remains a mainstay, given its central role in establishing a histologic diagnosis and in relieving symptoms of mass effect by mechanical cytoreduction. In addition, mounting clinical data reinforce the conventional notion that a greater extent of resection can improve outcomes and prolong life expectancy.
As is the case for all solid tumors, the advantages of surgical resection for glioma must also be balanced with the potential risks of operative morbidity, and, as such, a great deal of focus in neurosurgical oncology is placed on minimizing collateral damage to surrounding eloquent brain.
Central to performing minimally morbid surgery is a thorough understanding of neuroanatomy and physiology in the region of interest. Given that primary central nervous system (CNS) tumors are highly infiltrative, display variable gross appearance, and may incorporate functional brain, the measures and observations that are used to plan a surgical approach must be diverse and must possess great precision. To that end, functional brain mapping allows the pursuance of safer operations with more aggressive surgical resections; these techniques include “gold standard” procedures such as direct electrical stimulation as well as newer, less invasive imaging technologies that can be integrated into preoperative planning processes as well as intraoperative decision making.
The first region of the brain to be mapped was the motor cortex. Many strategies to refine surgical approaches have been developed to minimize damage to motor cortex and motor fibers. Although similar principles have since been applied to the preservation of sensory, language, and memory functions, the mapping of motor pathways in the context of intracranial malignancy is a unique entity and thus poses a distinct set of risks and challenges. As reviewed herein, the history and background of motor mapping techniques are discussed, along with the current state of functional motor mapping in neurosurgical oncology and the potential implications of complementary technologies on the surgical management of patients with glioma.
Early work on the motor cortex
Cerebral localization of function is one of the most fascinating and controversial topics in neurologic history. The first observation that control over motor function could be lateralized in the brain dates back to the fifth century bc , when the ancient Greek physician Hippocrates noted that unilateral cerebral injury results in contralateral paralysis. Over the next 2 millennia, there was little written to explain this phenomenon. Interest in cerebral physiology instead seemed to be fixated on a philosophic discussion of the brain as “the seat of the soul.” It was not until the early nineteenth century that this focus began to shift toward a more localized or segmental view of brain functions, largely due to theories of phrenology set forth by Franz Joseph Gall (1758–1828). Although Gall was heavily criticized by those who believed his work to be pseudoscience and damaging to religion, he was nevertheless instrumental in altering thinking in the field.
The latter part of the nineteenth century fostered a series of seminal clinical discoveries related to specific localization of function in the cortex, beginning with John Hughlings Jackson’s assertion in 1864 that “the convolutions of the brain must contain nervous arrangements representing movements.” In 1870, the German neurologist Gustav Fritsch and an anatomist Eduard Hitzig performed the first experiment demonstrating that topographically restricted electrical stimuli could be applied to the mammalian cerebral cortex to elicit corresponding contralateral movements. Although perhaps less well recognized, these findings were shortly followed by the first recorded experience with direct electrical stimulation of the human brain by Robert Bartholow in 1874, who, inspired by localized testing on animal brains by contemporary David Ferrier, attempted to elicit sensory and motor responses by applying wires to the exposed dura of a patient who had a hole in her skull caused by a cancerous ulcer.
The earliest maps that depicted specific localization of motor control in the human cortex were based on findings from Ferrier’s work with monkeys, in which recordings of movements in response to cortical stimulation were grossly transferred to an outline of the human brain. These maps, along with a map developed by the surgeon Horsley, which was also based on experiments on monkeys and limited observations in humans, were the first of their kind to be included in Gray’s Anatomy in 1887.
In 1901, Harvey Cushing, at an early stage in his career, began to map the primate motor cortex with Charles Sherrington and by 1909 had published numerous reports on his experience with intraoperative faradic stimulation in patients being operated under local anesthesia, thus confirming somatotopy of the human cortex along the precentral and postcentral gyri. Advances in functional cortical localization were also enhanced significantly by Otfrid Foerster, who, through close collaboration with Oskar and Cécile Vogt, developed a broader, more complex human cortical map from the observations he made during surgeries for patients with epilepsy. In 1928, Wilder Penfield traveled to Germany, which marked the beginning of his collaborative work with Foerster. Over the next decade, Penfield performed extensive intraoperative investigations that would ultimately shape the modern understanding of cortical organization; synthesizing data from more than 163 craniotomies, Penfield eventually simplified his findings in a proverbial homunculus cartoon to convey the relative cortical representation of various anatomic parts.
With a special emphasis on mapping, the pioneers in cortical localization tended to perceive the brain as a collection of discrete functional areas. The original observations of Sherrington and Cushing suggested that the motor cortex is delimited within a narrow precentral strip, a legacy that can still be appreciated in modern anatomy textbooks; however, this degree of localization has perhaps been overemphasized in spite of abundant scientific evidence to support the finding that sensorimotor function is in fact broader and has overlapping cortical representations. Regardless, over the past 150 years, the advancements made by forefathers in neurosurgery initiated an exponential increase in the understanding of cortical representations; the original mapping techniques used by Penfield and others, which involved continuous stimulation for 1 to 6 seconds with a 60-Hz line frequency, set a standard for performing intraoperative neurophysiologic examinations based on the electrical excitability of the human brain, and many original principles of electrocortical stimulation (ECS) remain largely unchanged in current practice.
Principles of direct cortical stimulation
The application of ECS as a tool for functional manipulation is based on the resting membrane potential of a neuron, which varies between −60 and −100 mV because of the asymmetrical distribution of charges across the lipid layers of the cell membrane. When the membrane is depolarized (in this case, via local application of an electrode), an action potential is generated. Membrane depolarization is a binary event that, once triggered, exhibits consistent characteristics regardless of the stimulation parameters.
The first and primary concern for ECS is safety, given that electrical stimulation to the cerebral parenchyma can lead to neural damage through multiple possible mechanisms. In addition to the direct effects of power dissipation (ie, heat) on surrounding tissues, prolonged application of electrical current may also result in the toxic accumulation of negative charge at the cathode and electrode dissolution products at the anode. To address this risk, biphasic impulses are recommended, which compensate for relative depolarization and hyperpolarization of pericathodic and perianodic tissues. In addition, rectangular impulses are used to offset the phenomenon of accommodation, which otherwise occurs when membrane potentials depolarize gradually.
Perhaps the greatest risk of ECS is the induction of seizure. After stimulation, neurons are transiently refractory for 0.6 to 2.0 minutes, after which they enter a short phase of hyperexcitability and are thus at greater risk for unintentional depolarization; in one study, prolonged 60-Hz stimulation produced clinical or subclinical seizures in more than 20% of patients. Most such events are minor or self-limiting, and some have found that the seizures can be terminated more quickly by application of cold Ringer lactate to the cortex. Furthermore, owing to its overall safety, it has been demonstrated that in the absence of epileptiform activity, repeated stimulation with impulses of the magnitude used for motor mapping do not induce permanent histologic changes in the human cortex, and functionality returns to normal levels almost immediately after removal of the stimulus.
Aside from safety, the most valuable characteristics for any method of ECS are reproducibility and precision. Electrical stimuli are characterized in detail by plotting their ability to elicit a tissue response (in this case, neuron excitability). The ideal stimulus, with regard to intensity and duration, at which an action potential is triggered with minimal energy input is termed chronaxis. Chronaxis, by definition, is the shortest duration of an effective electrical stimulus that is equal to twice the minimum strength required for neuronal excitation (rheobasis). These parameters are inherently dependent on the impedance of the tissue being stimulated, which in turn can vary significantly as a function of anesthesia and local pathologic condition, such as tumor.
Factors that affect the precision of depolarizing impulses and thus the spatial resolution of ECS include electrode size and spacing, the type of electrodes that are used, and the current level. Although 2 electrodes are always necessary for producing a current, stimulation is considered to be monopolar when a single electrode is localized to the target tissue and the second grounding electrode is placed at some distance from the site of stimulation. Although some assert that the placement of a monopolar electrode is physically unambiguous and thus more precise, pathway of the current from a monopolar source may be less predictable than that from the bipolar approach, in which both the cathode and anode are located close to the target tissue and evoked changes are confined to an elliptical area based on the electrodes. However, most recent evidence suggests that this perception of bipolar stimulation may not be completely accurate because the threshold of activation in the region of the cortex between electrodes has been demonstrated to be significantly greater than the areas directly beneath the anode and cathode. There is a relative dearth of literature directly comparing bipolar with monopolar stimulation; however, findings suggest that bipolar mapping is more sensitive in localizing functionality for certain areas such as the premotor frontal cortex. Despite evolving interest in the use of monopolar cortical stimulation for mapping, bipolar electrodes nonetheless remain standard and tend to be generally preferred in practice.
Current Density Distribution Generated by ECS
Despite the extensive use of ECS both clinically and experimentally, there are relatively few data available concerning what specific cells or parts of cells in the CNS are being activated. Although surgeons may generally have a greater interest in the behavioral consequences of stimulation, studies should be communicated in such a way to allow interpretation of findings on a cellular level. Because of uncertainties in tissue properties and geometry, the various numerical models that have been developed to describe the relationship between current density and distance from an electrode are expected to represent only crude predictions. Generally, it is thought that the amount of current applied to a given neuron is directly proportional to the square of the distance between the neuron and the electrode tip. Models that predict distribution of local potential are based on the solution of the Laplace equation:
where V is the scalar potential, σ is the conductivity, and ∇ is the gradient vector. Assuming uniform conductivity,
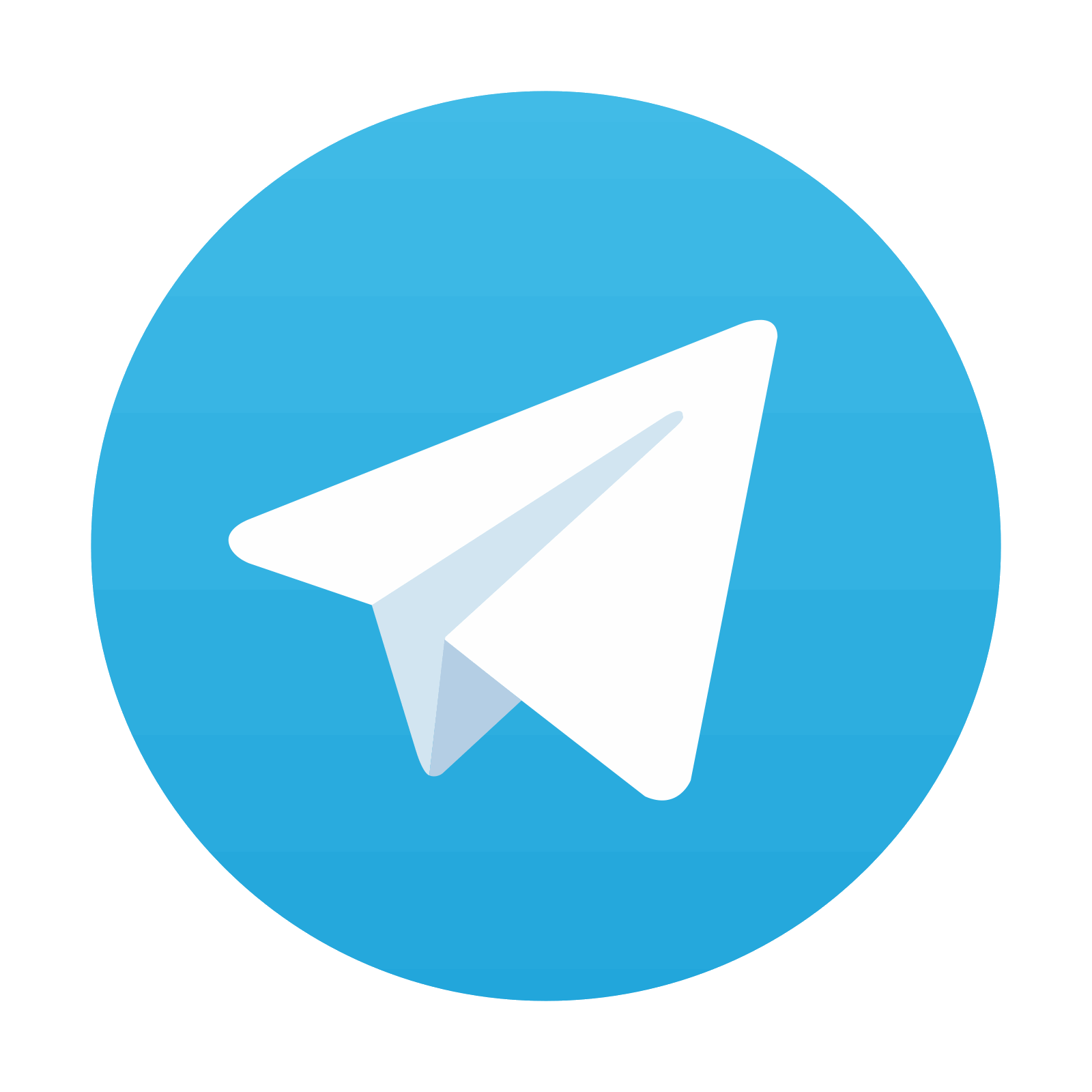
Stay updated, free articles. Join our Telegram channel
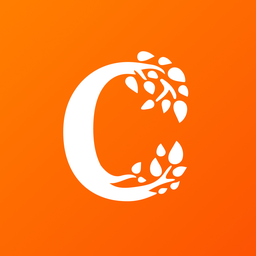
Full access? Get Clinical Tree
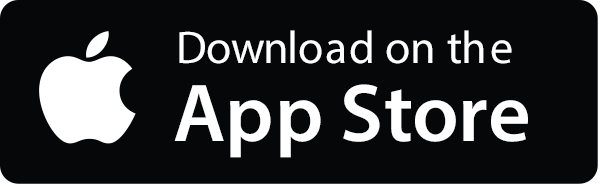
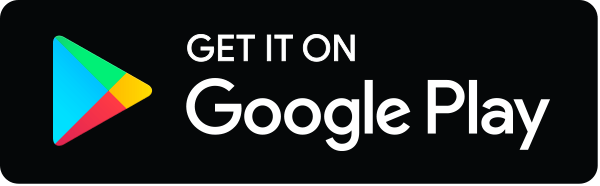