CLINICAL CASE | Homonymous Hemianopsia
A 70-year-old woman suddenly developed difficulty seeing on the left side. She was taken to the emergency room. She reported that when she looked directly at her husband, she only saw the right side of his face. On testing in the emergency room, she was found to have a homonymous hemianopsia field defect. The patient did not have left hemineglect or difficulty drawing shapes or in describing the spatial relationships between objects. Figure 7–1A is an MRI showing damage to the right medial occipital lobe and underlying white matter. This was produced by occlusion of the posterior cerebral artery distal to the thalamus.
Answer the following questions based on your reading of the chapter, inspection of the images, and consideration of the neurological signs.
1. Draw the visual field impairment.
2. Explain why the patient does not have macula sparing.
3. Why is it that the patient’s visual spatial aptitude is not impaired and that she does not have hemineglect?
Key neurological signs and corresponding damaged brain structures Homonymous hemianopsiaThis is loss of sight in the contralateral visual fields (see Figure 7–3). It can be due to lesion of the visual pathway proximal to the optic chiasm, on one side: along the optic tract, the lateral geniculate nucleus, optic radiations, and occipital lobe. The MRI shows a lesion of the medial occipital lobe. The primary and higher-order visual cortical areas are located here (see Figure 7–15). The basic visual loss is attributed to primary visual cortex damage.
Lack of macular sparingAfter occlusion of the occipital branch of the posterior cerebral artery, a distal artery supplying more selectively the visual cortex, the occipital pole may be relatively unaffected. This is because of collateral blood supply from the middle cerebral artery. Figure 7–1B shows this overlap schematically. In the patient, the infarction was not limited to the visual cortex gray matter; there was some involvement of the optic radiations.
Lack of hemineglect and preservation of visuo spatial aptitudeDamage to the region of the right posterior parietal and occipital lobes can produce hemineglect and disorders of visuospatial aptitude. This could be produced by occlusion/hemorrhage of superficial branches of the middle cerebral artery, which supplies the posterior parietal lobe, or the deep branches of these two arteries, which supply parts of the underlying white matter. These impairments are notably absent in this patient. This is because the lesion spares the posterior parietal lobe and the lateral occipital lobe.
As a species, humans depend more on vision than any other sensory modality. If strength in numbers is an indication of the importance of vision, then a simple axon count and an inventory of brain areas devoted to vision are very telling. The optic nerves, which connect the eyes with the visual processing centers of the brain, each contain about 1 million axons. The cerebral cortex, where visual messages from our eyes are analyzed and perceptions are formed, contains a bewilderingly large number of distinct areas—more than two dozen by some estimates—devoted to one or another aspect of visual processing.
In many ways, the visual system is organized like the somatic sensory systems, considered in Chapters 4 and 5. For instance, the topography of connections in the visual system is determined largely by how the receptive sheet is organized. In fact, these connections are so systematized and predictable that clinicians can use a visual sensory defect to pinpoint with remarkable precision the location of central nervous system damage. Another similarity is that both systems have a hierarchical and parallel organization. In a hierarchically organized system, distinct functional levels can be discerned with respect to one another, each with clear anatomical substrates. In vision, as in somatic sensation, multiple hierarchically organized pathways carry information from receptors to structures in the central nervous system. Each pathway processes visual information for a different purpose, such as perception of the shape, motion, and color of objects.
Visual perception, like perception for the other senses, is not a passive process; our eyes do not simply receive visual stimulation. Rather, the position of the eyes is precisely controlled to scan the environment and to attend selectively and orient to specific visual stimuli. In addition to the pathways from the retina to the cortex for perception, there is a separate pathway to the brain stem for controlling eye movements. This chapter begins with an overview of the visual pathways for perception and eye movement control. It then considers the structure and anatomical connections of the components of these pathways. Finally, the chapter examines how the clinician can, with remarkable precision, localize disturbances of brain function by using knowledge of the organization of the visual system. The visual path for eye movement control will be revisited when we consider the circuits for eye movement control (see Chapter 12).
The visual pathway that mediates perception and the pathway that controls eye movement originate in the retina, a thin sheet of neurons and glial cells that adheres to the posterior inner surface of the eyeball (Figure 7–2A). Also located here are the photoreceptors (Figure 7–2A, inset), which synapse on retinal interneurons that, in turn, synapse on ganglion cells. Ganglion cells are the retinal projection neurons, which synapse in the thalamus and brain stem. The axons of ganglion cells travel in the optic nerve (cranial nerve II), and ganglion cells from each eye contribute axons to the optic nerve on the same side. Some ganglion cell axons decussate in the optic chiasm (Figure 7–2A, B) en route to the thalamus and brain stem, whereas other axons remain uncrossed. Together the crossed and uncrossed ganglion cell axons, reordered according to a precise plan (see below), course in the optic tract (Figure 7–2B). Thus, each optic tract contains axons from both eyes.
FIGURE 7–2
Organization of the two visual pathways, the retinal-geniculate-calcarine pathway (A, B) and the pathway to the midbrain (A, C). The inset on the left shows the general organization of the retina. The photoreceptor transduces visual stimuli and transmits the sensory information, encoded in the form of nonpropagated potentials, to retinal interneurons. The interneurons transmit the visual information to the ganglion cells. Ganglion cell axons form the optic nerve once they exit the eyeball. A. Midsagittal view of the brain, showing visual paths to the thalamus and cortex and the path to the midbrain. B. Inferior brain view, showing the retinal-geniculate-calcarine pathway. C. Inferior brain view, showing the pathway to the midbrain.

The Pathway to the Primary Visual Cortex Is Important for Perception of the Form, Color, and Motion of Visual Stimuli
The principal thalamic target for ganglion cells is the lateral geniculate nucleus. This thalamic relay nucleus is analogous to the ventral posterior nucleus, the main somatic sensory relay nucleus. The lateral geniculate nucleus projects to the primary visual cortex via a pathway called the optic radiations. This projection is important for perception. It comprises multiple functional pathways, whose axons intermingle: one that is more important for perceiving the form of visual stimuli, another for color, and a third for the location and speed of motion of stimuli.
The primary visual cortex, or V1, is located in the occipital lobe, along the banks and within the depths of the calcarine fissure (Figure 7–2A). The primary visual cortex is also referred to as the striate cortex because myelinated axons (termed the stripe of Gennari) form a prominent striation. Efferent projections from the primary visual cortex follow one of three principal pathways. One pathway projects to the secondary and higher-order visual cortical areas in the occipital lobe (see Figure 7–16). The higher-order visual areas partially encircle the primary visual cortex. Each of these visual cortical areas is retinotopically organized. Whereas the primary visual cortex is important in visual signal processing that is fundamental to all aspects of visual perception, many of the higher-order cortical areas are each important in different aspects of vision. For example, the primary visual cortex processes information for stimulus form, color, and motion; one of the higher-order areas (V4) is important for color vision; and a different area is important for discriminating the direction and speed of a moving stimulus (V5; see Figure 7–15). The axons of the second path from the primary visual cortex decussate in the corpus callosum and terminate in the contralateral primary visual cortex. This projection is also for visual perception. It helps to unify images from the two eyes into a perception of a single visual world. Finally, the third path from the primary visual cortex descends to the visuomotor centers of the midbrain for focusing the images of interest on the retina and for moving the eyes to salient objects.
Certain retinal ganglion cells project to the midbrain directly (Figure 7–2A, C), principally to two structures: the superior colliculus and the pretectal nuclei. The ganglion cell axons traveling to the midbrain skirt the lateral geniculate nucleus and course in a tract named the brachium of superior colliculus (Figure 7–2B; see also Figure 7–9). The superior colliculus, found in the tectum of the midbrain (see Figure 2–9), is dorsal to the cerebral aqueduct (Figure 7–2C). In lower vertebrates, such as amphibians and birds, the superior colliculus is termed the optic tectum and is the principal brain structure for vision, in lieu of a visual cortex. In mammals and especially humans, the superior colliculus has a minimal role in perception but an important role in the control of saccades, rapid eye movements that quickly shift visual gaze from one object to another. The pretectal nuclei are rostral to the tectum, at the midbrain-diencephalic junction (see Figure 7–9). The pretectal nuclei participate in pupillary reflexes, which regulate the amount of light reaching the retina, as well as other visual reflexes (see Chapter 12).
Additional brain stem and diencephalic projections of the optic tract serve other functions. For example, one projection reaches midbrain nuclei that are important in the reflexive control of eye position to stabilize images on the retina when the head is moving. These nuclei comprise the accessory optic system. As another example, a retinal projection to the hypothalamus is important for circadian rhythms (see Chapter 15).
When the eyes are fixed straight ahead, the total area seen is called the visual field, the combined visual fields of each eye (Figure 7–3A). Although the visual field can be divided into right and left hemifields, the visual field of each eye is not simply one hemifield. Similar to when you look through binoculars, the field of view of each eye overlaps extensively. As a result, the visual field includes a central binocular zone (Figure 7–3A, dark shading), where there is stereoscopic vision, and two monocular zones (Figure 7–3A, lighter shading). Each hemifield is therefore seen by parts of the retina of each eye.
After light enters the eye through the cornea, the transparent avascular portion of the sclera, it is focused onto the retinal surface by the lens (Figure 7–4A). The lens inverts and reverses the visual image projected on the retina. When you look at an object, you move your eyes so that the object’s image falls upon the fovea, a specialized high-resolution portion of the retina. The fovea is centered within a morphologically distinct region of the retina called the macula lutea (Figure 7–4B). The brain precisely controls the position of the eyes to ensure that the key portion of an image falls on the fovea of each eye. A vertical line passing through the fovea divides the retina into two halves, a nasal hemiretina and a temporal hemiretina. Each hemiretina includes half of the fovea and the remaining perifoveal and peripheral portions of the retina. The anterior portions of the nasal hemiretinae correspond to the temporal crescents, which are monocular zones receiving visual information from the temporal parts of the visual fields (Figure 7–5).
FIGURE 7–4
A. Sagittal view shows the key features of the optical properties of the eye. B. Course of ganglion cell axons along the surface of the retina and into the optic nerve at the optic disk. (B, Adapted from Patten H. Neurological Differential Diagnosis. 2nd ed. New York, NY: Springer-Verlag; 1998.)

FIGURE 7–5
Schematic horizontal view of the eyes looking toward a person, showing the location of the visual fields for each eye and how information projects on the two retinae. The left and right visual fields are shown in blue and green; overlapping regions are striped. For the left eye, visual information from the dark blue region falls on the left temporal hemiretina, and from the light blue region, on the left nasal hemiretina. For the right eye, visual information from the dark green region falls on the right temporal hemiretina, and from the light green region, on the right nasal hemiretina.

Consider the relationship between an object being viewed and where its image falls on the retina (Figure 7–5). When you look at someone’s face and, for example, fixate on the person’s nose, the left side of the face is within the left visual hemifield. The image of the left side falls on the nasal hemiretina of the left eye and the temporal hemiretina of the right eye. Although each eye views the entire face, visual information from the visual hemifield on one side is processed by the visual cortex on the opposite side (see below).
Figure 7–4B also shows the optic disk, where retinal axons leave the eyeball and the blood vessels serving a part of the retina enter and leave the eye. This corresponds to the blind spot (Figure 7–3B) because the optic disk has no photoreceptors. Interestingly, an individual is not aware of his or her own visual blind spot until it is demonstrated. The fovea and optic disk can be examined clinically using an ophthalmoscope to peer into the back of the eye.
The retina is a laminated structure, as revealed in a section oriented at a right angle to its surface (Figure 7–6). Other components of the visual system also have a laminar organization. Lamination is one way the nervous system packs together neurons with similar functions and patterns of connections. The spatial reference point for describing the location of the different layers is the three-dimensional center of the eye. The inner, or proximal, retinal layers are close to the center of the eye; the outer, or distal, layers are farther from the center (Figure 7–6, inset).
Although the retina has many anatomically distinct layers (Figure 7–6), the cell bodies of most retinal neurons are located within three layers. This can be best observed in a micrograph of the retina of the mouse (Figure 7–7), in which the various cell types can be identified genetically or immunohistochemically.
FIGURE 7–7
At the fovea, retinal interneurons and ganglion neurons are displaced so that light falls directly onto the photoreceptors (top). A cross-section through the mouse retina showing lamination of cell bodies and synapses (bottom left). Photoreceptors are stained purple-blue (using an antibody to cone arrestin). Amacrine and ganglion cells are stained red (calcium binding protein calbindin). Bipolar cells are green. (Micrograph courtesy of Dr. Rachel Wong, University of Washington. Schematic diagram showing major cell types and connections in the vertebrate retina. Adapted from Dowling JE, Boycott BB. Organization of the primate retina: electron microscopy. Proc R Soc Lond B. 1966;166:80-111.)

The cell bodies of the two classes of photoreceptors—rods, for night vision, and cones, for high-acuity daylight vision—are located in the outer nuclear layer.
The cell bodies and many dendritic processes of retinal interneurons—bipolar, horizontal, and amacrine cells—are located in the inner nuclear layer.
Ganglion cells, the retinal projection neuron, are located in the innermost retinal cell layer, the ganglion cell layer.
Cones contain the photopigments for color vision and come in three different classes according to their absorption spectra: red, green, or blue. Cone density is highest at the fovea and decreases continuously to the peripheral retina. This is why visual acuity is greatest at the foveal and decreases to the peripheral retina. Rods contain the photopigment rhodopsin and are optimally suited for detecting low levels of illumination, such as at dusk or at night. In fact, a single photon can activate a rod cell. Rods are absent in the fovea and are densest along an elliptical ring in the perifoveal region, which is the location of maximal light sensitivity. This helps to explain why, when discerning a faint object at night, we do best by looking off to one side, rather than directly at it.
Bipolar cells connect photoreceptors directly with the ganglion cells (Figure 7–7). Of the two principal classes of bipolar cells, cone bipolar cells and rod bipolar cells, the former receive synaptic input from a small number of cone cells to give high visual acuity and color vision. By contrast, rod bipolar cells receive convergent input from many rods for less visual acuity but increased sensitivity to low levels of illumination. The actions of horizontal cells and amacrine cells enhance visual contrast through interactions between laterally located photoreceptors and bipolar cells. Horizontal cells are located in the outer part of the inner nuclear layer, whereas amacrine cells are found in the inner portion. Many amacrine cells contain dopamine, which plays a role in adapting retinal synaptic activity to the dark.
There are two major classes of retinal ganglion cells—M and P cells. The M (or magnocellular) cell has a large dendritic arbor, enabling it to integrate visual information from a wide portion of the retina. M cells are thought to play a key role in the analysis of stimulus motion as well as gross spatial features of a stimulus. The P (or parvocellular) cell, with its small dendritic arbor, processes visual information from a small portion of the retina. These cells are color sensitive and are important for discriminative aspects of vision, such as distinguishing form and color. Ganglion cell axons collect along the inner retinal surface (Figures 7–4B, 7–6, and 7–7) and leave the eye at the optic disk (Figure 7–4B), where they form the optic nerve.
Connections between many retinal neurons are also made within specific laminae (Figure 7–7). Connections between photoreceptors and retinal interneurons are in the outer synaptic (or plexiform) layer. Bipolar cells synapse on ganglion cells in the inner synaptic (or plexiform) layer.
The cellular organization of the retina might seem unexpected because light must travel through retinal layers that contain axons, projection neurons, and interneurons to reach the photoreceptors. The consequences of this organization on visual acuity are minimized by an anatomical specialization at the fovea. Here the retinal interneurons and ganglion cells are displaced, exposing the photoreceptors directly to visual stimuli and optimizing the optical quality of the image (Figure 7–7, inset). Moreover, ganglion cell axons are unmyelinated while they are in the retina, which increases the transparency of the retina and facilitates light transmission to the photoreceptor layer. Ganglion cell axons become myelinated once they enter the optic nerve. Since the retina develops from the central nervous system, the myelin sheath surrounding ganglion cell axons is formed by oligodendrocytes (see next section).
There are important nonneural cells in the retina. Müller cells, a kind of astrocyte, have important structural and metabolic functions. Their nuclei are located in the inner nuclear layer, and their processes stretch vertically across most of the retina (Figure 7–6). The other nonneural element associated with the retina, the pigment epithelium, is external to the photoreceptor layer (Figure 7–5A) and serves metabolic and phagocytic roles. For example, cells in the pigment epithelium help remove rod outer segment disks that are discarded as part of a normal renewal process. Because the retina does not tightly adhere to the pigment epithelium, it can become detached following a blow to the head or eye. This results in a partially detached retina and loss of vision in the detached portion.
The circulation of the retina has a dual organization. The arterial supply of the inner retina is provided by branches of the ophthalmic artery, which is a branch of the internal carotid. The outer retina is devoid of blood vessels. Its nourishment derives from arteries in the choroid, the layer of ocular tissue between the inner retina and the outer sclera. This may be why the photoreceptors are in the outer retina.
The optic nerve is cranial nerve II, but it is actually a central nervous system pathway rather than a peripheral nerve. This is because the retina develops from a displaced portion of the diencephalon, rather than from neural crest cell, as primary somatic sensory neurons. The optic nerves from both eyes converge at the optic chiasm (Figure 7–8). The axons of ganglion cells of each nasal hemiretina decussate in the optic chiasm and enter the contralateral optic tract, whereas those of each temporal hemiretina remain on the same side and enter the ipsilateral optic tract (Figure 7–8). Thus, each optic tract contains axons from the contralateral nasal hemiretina and the ipsilateral temporal hemiretina (Figure 7–8). Despite the incomplete decussation of the optic nerves in the chiasm, there is a complete crossover of visual information: Visual stimuli from one half of the visual field are processed within the contralateral thalamus, cerebral cortex, and midbrain.
FIGURE 7–8
Horizontal view of the visual system, showing the portions of the retina of each eye that receive information from the left visual field. Ganglion neuron axons from the nasal hemiretinae decussate; those from the temporal hemiretinae project to the brain ipsilaterally. A. View of the base of the skull showing the regional anatomy of the optic chiasm. B. Paths taken by ganglion cell axons from the different parts of the retinae of each eye. (B, Adapted from Patten H. Neurological Differential Diagnosis. 2nd ed. New York, NY: Springer-Verlag; 1998.)
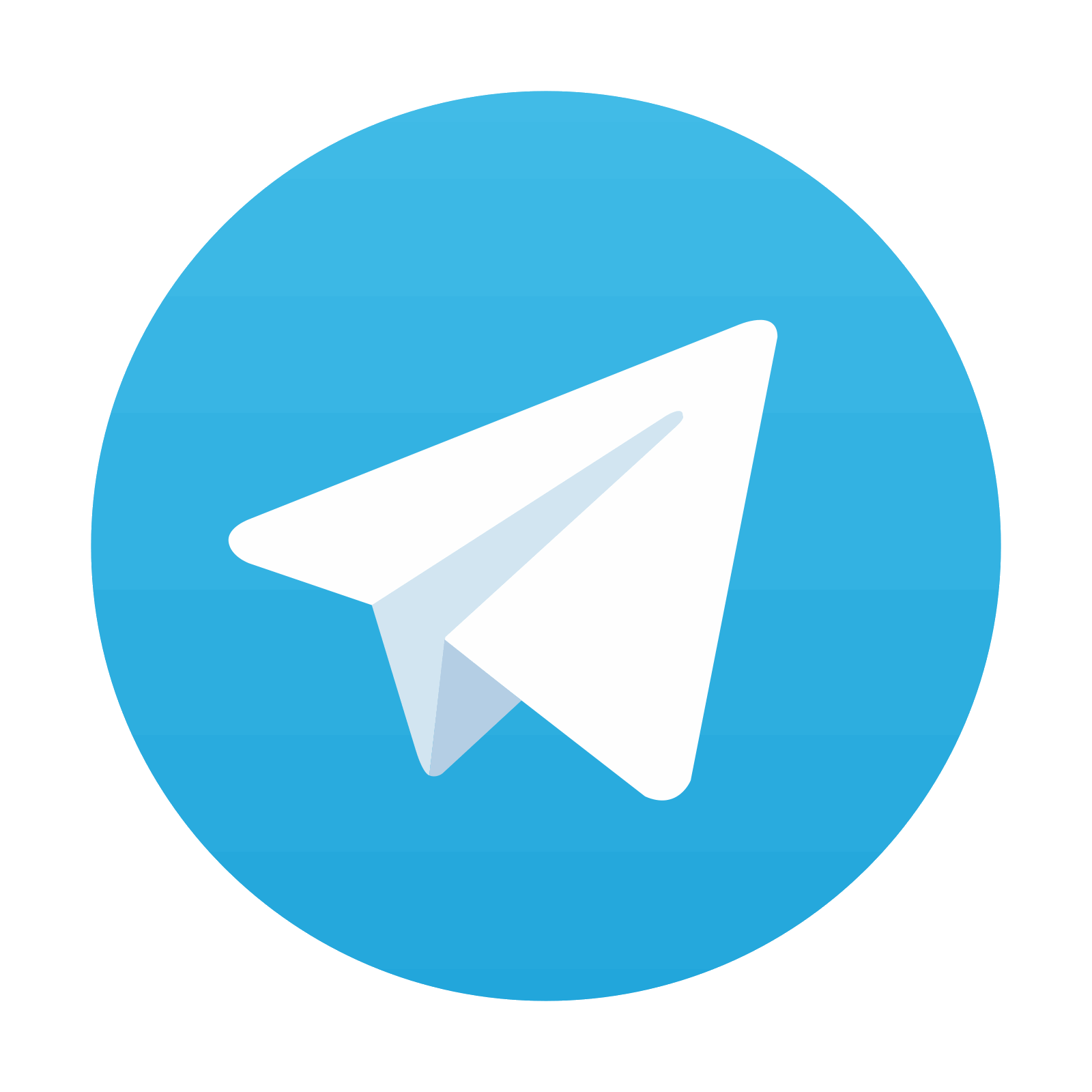
Stay updated, free articles. Join our Telegram channel
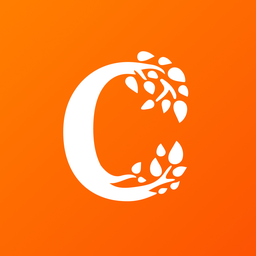
Full access? Get Clinical Tree
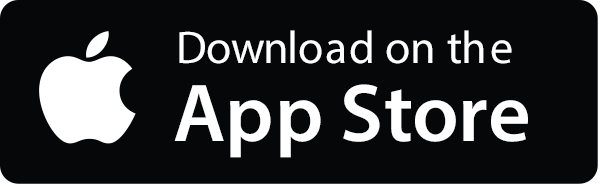
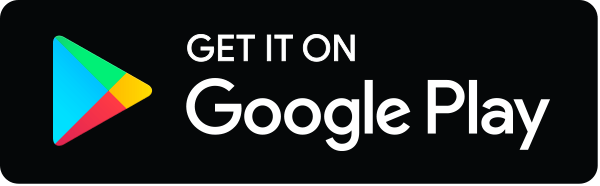
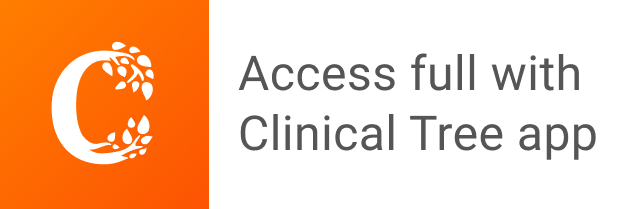