Figure 20-1. Cross section of the human eye. The gray arrow shows the path of light through the optical apparatus.
A variety of conditions may affect the cornea and, by disturbing the transmission of light through the cornea, degrade the quality of the visual image. Damage to the cornea may occur in chronic infections of herpesvirus, of Chlamydia trachomatis, or of the bacteria that cause conjunctivitis. Trauma may, of course, cause permanent damage to the cornea. In addition, various metabolic diseases may result in crystal deposits or other opacities in the cornea.
Chambers of the Eye
Just behind the cornea is the fluid-filled anterior chamber, which is bound posteriorly by the iris and the opening of the pupil (Fig. 20-1). A second fluid-filled space, the posterior chamber, is bound anteriorly by the iris and posteriorly by the lens and its encircling suspensory ligament (zonule fibers). Fluid is continuously produced by the epithelium over the ciliary body around the rim of the posterior chamber and flows through the pupillary opening into the anterior chamber. It then drains into a set of modified veins, the canals of Schlemm, that are located around the rim of the anterior chamber in the angle where the iris meets the cornea. Because the suspensory ligament encircling the lens consists of discrete strands, the fluid in the posterior chamber is in contact with the vitreous body, the gelatinous mass that fills the main space of the eyeball between the lens and the retina.
Any condition that obstructs the outflow of fluid via the canals of Schlemm can lead to glaucoma, a buildup of fluid and hence pressure in the entire eyeball, with resultant damage to the retina and optic nerve and eventual blindness. Patients with glaucoma often comment that their vision is blurred but not dimmed. A normal amount of light reaches the retina, but the progressive loss of photoreceptors causes blurring of the visual image. Damage proceeds from the periphery of the retina toward the central region where the fovea is located. In most cases of glaucoma (about 90%), the angle between the iris and cornea is normal (open-angle glaucoma), and the cause of the increase of pressure is unknown. In about 5% of patients, the angle between cornea and iris is abnormally acute (closed-angle glaucoma) and blocks the normal flow of fluid. In the remaining cases, the canals of Schlemm are blocked by debris from infection, complications of diabetes, or hemorrhage into the anterior chamber. When the intraocular pressure is above 20 mm Hg, optic nerve damage is a concern. The resulting visual loss proceeds from partial (in the periphery first) to total.
Iris
The iris is a pigmented structure lying directly anterior to the lens (Fig. 20-1). The connective tissue, or stroma, of the iris contains melanocytes that reflect or absorb light to give the iris its characteristic color. Also embedded in the stroma are the circumferentially organized sphincter muscle of the iris and the radially arranged dilator muscle (Fig. 20-1).
The innervation of the iris sphincter, which closes the pupil, is parasympathetic. This pathway begins with preganglionic neurons whose cell bodies lie in the Edinger-Westphal preganglionic nucleus and whose axons terminate in the ciliary ganglion. Axons of postganglionic ciliary ganglion neurons, in turn, end as neuromuscular synapses on the sphincter muscle and release acetylcholine. When activated, this pathway results in a reduction in pupil diameter, or miosis.
The innervation of the iris dilator, which opens the pupil, is sympathetic. The pathway begins with preganglionic neurons whose cell bodies lie in the intermediolateral cell column of the spinal cord at upper thoracic levels and whose axons terminate in the superior cervical ganglion. Axons of postganglionic superior cervical ganglion neurons, in turn, end as neuromuscular synapses on the dilator muscle and release norepinephrine. When activated, this pathway results in an increase in pupil diameter, or mydriasis. This phenomenon is a measure of the general state of the sympathetic tone. Anger, pain, or fear may result in an enlargement of the pupil in the absence of a change in lighting conditions. The pupillary light reflex, a contraction of the pupil in response to light, is used to assess the function of the nervous system, particularly at midbrain levels (see Chapter 28).
The circumference of the pupillary margin changes by a factor of six. This proportional change in muscle length is greater than any other in the human body. To accomplish this change, acetylcholine is released onto both the sphincter and dilator muscles. The effect is to activate muscarinic receptors that depolarize sphincter muscle cells and cause contraction. In addition, acetylcholine released by collaterals onto the dilator muscle mediates presynaptic inhibition of norepinephrine release and blocks dilator contraction. Thus, as the sphincter contracts, the dilator relaxes, strengthening the pupillary response to light.
Lens
The lens is a clear structure that focuses light on the retina (Fig. 20-1). The lens of the eye is a simple convex lens that inverts and reverses the image on the retina. Mechanisms that change the curvature of the lens are discussed in detail in Chapter 28.
Beginning at about the age of 40 years, the lens begins to lose its elasticity so that the shape it adopts when relaxed is more flattened than earlier in life. This change reduces the affected person’s ability to focus on near objects, a condition called presbyopia. Reading or bifocal corrective lenses are prescribed to aid the patient in performing tasks requiring close, detailed vision.
Cataract
Opacities in the lens, known as cataracts, are relatively common and can be seen as a cloudiness of the lens. Cataracts may be caused by congenital defects (e.g., secondary to maternal infection with rubella), persistent exposure to ultraviolet light, diabetes, high doses of some medications, radiation therapy, or poorly understood mechanisms that occur in aging. Current therapy consists of replacement of the lens with an inert plastic prosthesis, restoring sight but with a concomitant loss of accommodation.
Uvea
The iris, ciliary body, and choroid make up the vascular tunic of the eye, also called the uvea. The choroid is a highly vascularized, pigmented tissue layer lying between the retinal pigment epithelium and the sclera, the tough outer coating of the eye. Uveitis is an inflammation of these structures, often secondary to eye injury.
NEURAL RETINA AND PIGMENT EPITHELIUM
The inner surface of the posterior aspect of the eye is covered by the retina, which is composed of the neural retina and the retinal pigment epithelium (Fig. 20-2). In describing the layers and cells of the retina, it is common to use the terms inner and outer. Inner refers to structures located toward the vitreous (i.e., the center of the eyeball), whereas outer is used in reference to structures located toward the pigment epithelium and choroid.
Figure 20-2. Cells and layers of the retina. Photoreceptors (rods, r, and cones, c) are shown in green. Horizontal cells (h, gray) and bipolar cells (b, blue) receive input from photoreceptors; the bipolar cells, in turn, synapse onto amacrine cells (a, white) and ganglion cells (g, red).
The retinal pigment epithelium is a continuous sheet of pigmented cuboidal cells bound together by tight junctions that block the flow of plasma or ions. Its functions are as follows: (1) it supplies the neural retina with nutrition in the form of glucose and essential ions; (2) it protects retinal photoreceptors from potentially damaging levels of light; and (3) it plays a key role in the maintenance of photoreceptor anatomy via phagocytosis.
The neural retina contains the photoreceptors and associated neurons of the eye and is specialized for sensing light and processing the resultant information. The photoreceptors absorb quanta of light (photons) and convert this input to an electrical signal. The signal is then processed by retinal neurons, as discussed further on. Finally, the retinal neurons called ganglion cells send the processed signal to the brain via axons that collectively form the optic nerve.
The contact between the neural retina and the pigment epithelium is the adult remnant of the ventricular space of the developing eye cup. As such, it is mechanically unstable. This instability is demonstrated in a retinal detachment, in which the neural retina tears away from the pigment epithelium. Retinal detachment is more commonly seen in male patients and may result from a range of factors, such as trauma to the orbit or head, sequela to cataract surgery, genetic predisposition, degenerative diseases of the eye or optic nerves, and aging. Because photoreceptors are metabolically dependent on their contact with pigment epithelial cells, a detached retina must be repaired to avoid further damage. The detached part of the neural retina is reattached to the pigment epithelium by surgical procedures. The degree of functional recovery in the reattached part of the retina depends on its location. It also depends on how soon the reattachment is performed after the injury.
The neural retina has seven characteristic layers (Fig. 20-2). From outer to inner they are (1) a layer containing the photoreceptor cell outer and inner segments; (2) an outer nuclear layer, consisting of the nuclei of photoreceptor cells; (3) the outer plexiform layer, consisting of the synaptic connections of photoreceptors with second-order retinal cells; (4) the inner nuclear layer, containing somata of second-order and some third-order retinal cells; (5) the inner plexiform layer, another area of synaptic contact; (6) the ganglion cell layer, containing the cell bodies of the ganglion cells; and (7) the nerve fiber layer (or optic fiber layer), composed of the axons of the ganglion cells. These axons converge at the optic disc to form the optic nerve. Layers 2 through 7 are flanked by a pair of limiting membranes, which consist of glial cell processes joined by tight junctions. The outer limiting membrane is located between layers 1 and 2, and the inner limiting membrane is located between the nerve fiber layer and the vitreous.
The photoreceptor outer segments interdigitate with the melanin-filled processes of pigment epithelial cells (Fig. 20-2). These processes are mobile, and they elongate into the pigmented layer when the light is bright (photopic conditions) and retract when the light is dim (scotopic conditions). This mechanism combines with contractions of the iris to protect the retina from light conditions that would otherwise damage the photoreceptors. The iris, pigment epithelium, and circuitry of the retina all contribute to the eye’s ability to resolve the visual world over a wide range of light conditions.
The blood supply of the neural retina arises from branches of the ophthalmic artery; these branches are the central artery of the retina and the ciliary arteries. The central artery is usually the first branch of the ophthalmic, passes rostral and inferiorly adjacent to the dural sheath, and then enters the sheath and the optic nerve about 1.25 cm caudal to the bulb of the eye. After entering the eyeball, it branches out from the optic nerve head to serve inner portions of the neural retina. The ciliary arteries penetrate the sclera around the exit of the optic nerve and feed the choriocapillaris (a portion of the choroid), which in turn provides nutrients to the outer portions of the neural retina.
PHOTORECEPTOR CELLS
The rods and cones of the retina are responsible for photoreception, the process by which photons are detected and the information is transduced into an electrochemical signal. There are two basic types of photoreceptors, rods and cones (Figs. 20-3 and 20-4), although a special class of ganglion cell (melanopsin-containing ganglion cell) may be considered a third type of photoreceptor (see later). Rods and cones have the same general overall design. Light is detected and transduced in an outer segment that points toward the pigment epithelium. A narrow stalk, the cilium, connects the outer segment to a second expanded region called the inner segment, which contains mitochondria and produces the energy that maintains the cell. The cilium contains nine pairs of microtubules emanating from a basal body located in the inner segment. The nucleus and perikaryon of the cell are found in the outer nuclear layer; finally, the cell terminates in the outer plexiform layer in an expansion that makes synaptic contacts with neurons. This synaptic expansion is called the spherule in rod cells and the pedicle in cone cells. Both rod and cone synaptic terminals contain a characteristic dark sheet of protein called the synaptic ribbon. This structure may act as a “conveyor belt,” organizing vesicular release of transmitter.
Figure 20-3. The rod photoreceptor and the physiologic and chemical changes that occur in response to light. Events associated with light are shown in red. cGMP, cyclic guanosine monophosphate; 5′GMP, 5′-guanosine monophosphate; PDE, phosphodiesterase.
Figure 20-4. The cone photoreceptor (A). Cones, like rods, reduce their levels of neurotransmitter release when stimulated by photons. Cones and rods are also distinguished by prominent electron-dense synaptic ribbons in their terminals (B).
Rods
Rod cells are named for the shape of their outer segment, which is a membrane-bound cylinder containing hundreds of tightly stacked membranous discs (Fig. 20-3). The rod outer segment is a site of transduction. Photons travel through cells of the neural retina before striking the membranous discs of the rod outer segment. Molecules of rhodopsin within these membranes undergo a conformational change and along with transducin and phosphodiesterase induce biochemical changes in the rod outer segment, which reduce levels of cyclic guanosine monophosphate (cGMP). In the dark, cGMP levels in the rod outer segment are high. This cGMP mediates a standing sodium current. At rest, in the dark, sodium ions flow into the rod outer segment. This high resting level of sodium permeability results in a relatively high resting potential for rod cells, about −40 mV. These sodium channels of the outer segment membrane, which are normally open, close in response to increased calcium or a reduction in cGMP. This drives the membrane potential away from the sodium equilibrium potential and toward the potassium equilibrium potential, and the rod cell is hyperpolarized in response to a light stimulus (Fig. 20-3). Note that photoreceptors are the only sensory neurons that hyperpolarize in response to the relevant stimulus.
The hyperpolarization of the rod outer segment propagates passively (i.e., without firing an action potential) through the perikaryon to the rod spherule. In the absence of light, the photoreceptor terminals constantly release the transmitter glutamate at these synapses. The arrival of a light-induced wave of hyperpolarization causes a transient reduction in this tonic release of glutamate. As explained further on, this event can depolarize some of the cells that receive synapses from photoreceptor terminals while hyperpolarizing others.
Rhodopsin molecules are capable of a huge but finite number of photoisomerization events. Rather than replace individual rhodopsin molecules, every morning the distal one tenth of the outer segment is broken off and phagocytosed by the pigment epithelium. Through this process of rod shedding, the outer segment is constantly renewed. New discs are formed at the base of the outer segment and move outward so that the shed discs are replaced. In this way, the rod remains a constant length and the outer segment is renewed about every 10 days.
Cones
Like rod outer segments, cone outer segments also consist of a membranous stack (Fig. 20-4). Unlike in rods, however, these stacks of cone membranes are of constantly decreasing diameter (from cilium to tip), giving the cell its characteristic shape. Also, they are not enclosed within a second membrane but are open to the extracellular space adjacent to the pigment epithelium (Fig. 20-4).
The process of transduction in cones is generally similar to that in rods. Cone opsin absorbs photons and undergoes a conformational change, resulting in a hyperpolarization of the cell membrane (Fig. 20-4). This hyperpolarization propagates passively to the cone’s synaptic ending, the cone pedicle, in the outer plexiform layer. Cone pedicles and rod spherules both contain synaptic ribbons surrounded by vesicles, but cone pedicles are larger (Fig. 20-4). Serial-section electron microscopy has shown that the synaptic ribbons are actually a single extensive sheet of protein. Like rods, cones release the neurotransmitter glutamate tonically in the dark and respond to light with a decrease in glutamate release.
There are three types of cones, each tuned to a different light wavelength (Fig. 20-5). L-cones (red cones) are sensitive to long wavelengths, M-cones (green cones) to medium wavelengths, and S-cones (blue cones) to short wavelengths. Because any pure color represents a particular wavelength of light, each color will be represented by a unique combination of responses in the L-, M-, and S-cones.
Figure 20-5. Absorption spectra of rods and the three types of cones. Because the three cone spectra are different but overlapping, any wavelength of light in the visual spectrum (bottom scale) will elicit a set of response intensities in the three types of cones that is different from the set elicited by any other wavelength. Therefore any color in the visual spectrum can be uniquely encoded. The rod spectrum is shown for comparison even though rod input is not used in color recognition. Dim red light can be used to adapt humans to maximum rod sensitivity because red light (620 to 700 nm) is not absorbed by rods to any significant extent.
If one of these cone types is absent because of a genetic defect in the corresponding opsin, the affected person will confuse certain colors that look different to visually normal individuals and is said to be color blind. It is better, however, to think of this condition as “color confusion” because the patient can still see all colors of the visible spectrum; it is the ability to distinguish certain colors that has been lost. Because the genes for the L-cone (red-absorbing) and M-cone (green-absorbing) opsins are located on the X chromosome, color blindness is more common in men. Alteration of the gene for the S-cone (blue-sensitive) pigment, which is located on an autosome, is much rarer. The inability to detect a pure red is known as protanopia, and inability to detect green is known as deuteranopia.
Macula and Fovea
At the posterior pole of the eye is a yellowish spot, the macula lutea, the center of which is a depression called the fovea centralis (Fig. 20-6). Near the fovea, the inner retinal layers become thinner and eventually disappear so that at the bottom of the foveal pit, only the outer nuclear layer and photoreceptor outer segments remain. This allows a maximum amount of light to reach the photoreceptors with optimal fidelity.
Figure 20-6. Scanning electron micrographs of the primate fovea centralis (A) and of inner and outer segments of photoreceptors (B), mostly rods, in more peripheral areas of the retina. Only cones are present in the foveal pit. The surface striations (A) are ganglion cell axons en route to the optic nerve head. (Photographs courtesy of Dr. Bessie Borwein. From Borwein B: Scanning electron microscopy of monkey foveal photoreceptors. Anat Rec 205:363-373, 1983, with permission of Wiley-Liss, Inc.)
Most of the visual input that reaches the brain comes from the fovea. Cones, which are responsible for color vision, are the only type of photoreceptor present in the fovea. In contrast, rods, which are most sensitive at low levels of illumination, are the predominant photoreceptors in the periphery of the retina. The visual world is a composite formed from a succession of foveal images carrying form and color information supplemented with input from the peripheral retina carrying motion information.
RECEPTIVE FIELDS
The receptive field of a visually responsive neuron is defined as that region of the visual world in which a stimulus of the proper characteristics will influence the activity of the neuron. The influence may be either excitatory or inhibitory. Some neurons will exhibit an excitatory influence when the stimulus is in one location and an inhibitory influence when the stimulus is in a nearby location. The receptive field of a neuron is the sum of the areas in which the stimulus affects the activity of that neuron.
In the early stages of visual information processing, receptive fields have a characteristic concentric center-surround organization. The receptive field is roughly circular (Fig. 20-7). Stimuli in the center of this circle tend to evoke one type of response (e.g., depolarization), whereas stimuli in the doughnut-shaped outer rim evoke the opposite response (e.g., hyperpolarization). In later stages of visual processing, in the visual cortex, receptive field properties are more complicated.
Figure 20-7. How center-surround receptive fields are built in the visual system. Inputs from both receptors and horizontal cells contribute to the characteristic center-surround receptive fields of bipolar cells. A sign-conserving synapse is one in which hyperpolarization in the presynaptic cell promotes hyperpolarization in the postsynaptic cell. A sign-inverting synapse is one in which hyperpolarization in the presynaptic cell promotes depolarization in the postsynaptic cell. The example illustrated is of an “on” bipolar cell.
PROCESSING OF VISUAL INPUT IN THE RETINA
Among retinal cells, only retinal ganglion cells have voltage-gated sodium channels on their axonal membranes. As a result, only ganglion cells use action potentials to carry information. So-called calcium spikes resulting from an increase in calcium permeability are seen in amacrine cells. All other retinal cells use only graded potentials to process information.
The receptive field properties of each retinal cell depend on the processing of information passing through the neurons between the photoreceptor and the retinal cell in question. For example, a bipolar cell’s response is directly related to the activity of photoreceptors and horizontal cells (Figs. 20-7 and 20-8). As with all sensory systems, the structural, electrical, and synaptic properties of the cell are reflected in receptive field properties.
Figure 20-8. How ganglion cell receptive fields are built in the visual system. Both “on” and “off” bipolar cells contribute to the formation of receptive fields (A) in ganglion cells. Amacrine cells add information about transience (i.e., how long since the light has changed from on to off). X- or P-type ganglion cells respond linearly to the sine wave grating; the frequency of action potentials rises and falls in synchronization with the sine wave of light intensity used to stimulate them (B). When light strikes both center and surround, there is no net change in ganglion cell activity in either cell type. On the other hand, Y- or M-type ganglion cells respond best to the changes between light onset and offset. X- or P-type ganglion cells are also responsive to color (C). Two examples are shown, called R−G+ (for red inhibitory center and green excitatory surround) and R+G−. There are also blue-yellow combinations. IPL, inner plexiform layer.
Outer and Inner Plexiform Layers
Synaptic contacts in the retina are concentrated in the outer and inner plexiform layers (Fig. 20-2). The outer plexiform layer contains synapses among and between retinal photoreceptors, horizontal cells, and bipolar cells. Contacts between a single cone pedicle or rod spherule, a centrally placed postsynaptic bipolar cell process, and two laterally placed horizontal cell processes form a triad.
The inner plexiform layer contains synaptic contacts among and between bipolar, amacrine, and ganglion cells. In this layer, “off” and “on” bipolar cells terminate, making synaptic contact with the corresponding type ganglion cell. Amacrine cells also synapse with ganglion cells, other amacrine cells, and bipolar cells.
Horizontal Cells
Horizontal cells consist of a cell body and its associated dendrites and an axon that courses parallel to the plane of the retina to nearby and distant photoreceptors (Fig. 20-2). These cells receive glutaminergic input from photoreceptors and in turn form GABAergic synaptic contacts on adjacent rods and cones. Horizontal cells play a critical role in establishing the concentric center-surround receptive field organization of retinal ganglion cells (Figs. 20-7 and 20-8; see also Fig. 20-22A). They also mediate a process termed lateral inhibition that is responsible for sharpening the edges of images perceived in the visual system.
Bipolar Cells
In their position between photoreceptor cells and ganglion cells, bipolar cells help form a straight-through pathway for visual input (Fig. 20-2). Cone bipolar cells and rod bipolar cells are differentiated on the basis of their principal synaptic inputs.
Bipolar cells are the comparators, or edge detectors, of the retina. With the horizontal cells, they compare the activity in each region of the visual field with that in a nearby location. They are the first visual cells to exhibit the center-surround receptive field organization. In terms of physiologic response, there are two basic types of bipolar cells. On, or depolarizing, cells respond to a light stimulus in the receptive field center by depolarizing, whereas off, or hyperpolarizing, bipolar cells have the opposite center response (Figs. 20-7 and 20-8).
Imagine a series of photons striking a photoreceptor outer segment. Recall that the photoreceptor is hyperpolarized in response to light input, so that release of its neurotransmitter, glutamate, is decreased in the presence of light.
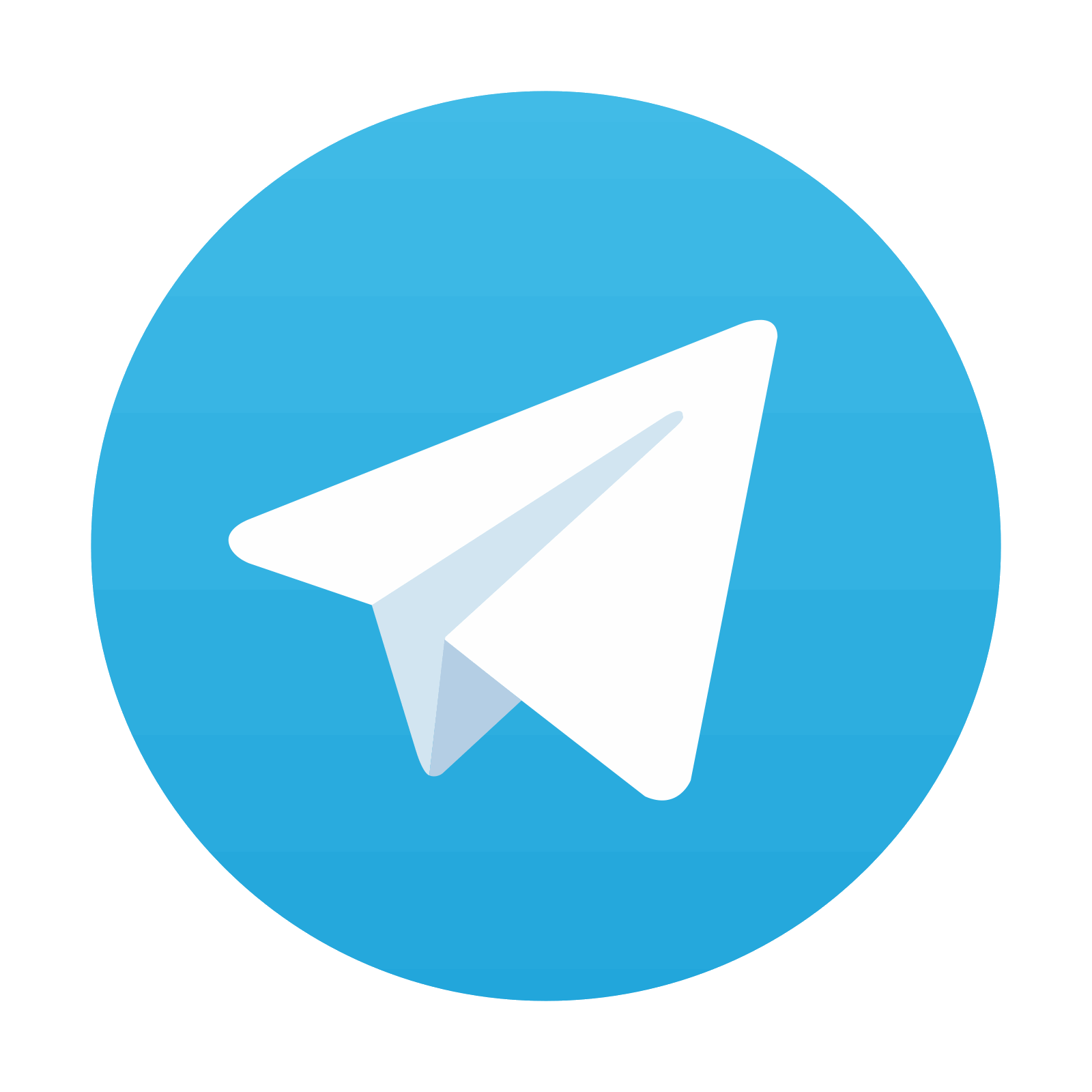
Stay updated, free articles. Join our Telegram channel
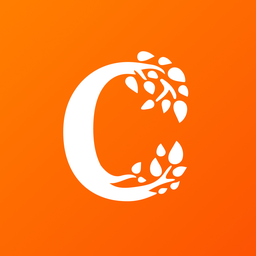
Full access? Get Clinical Tree
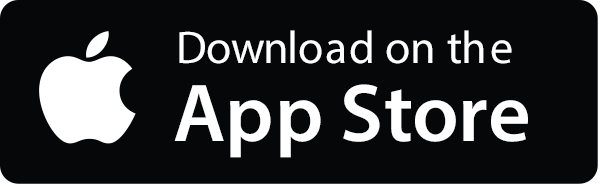
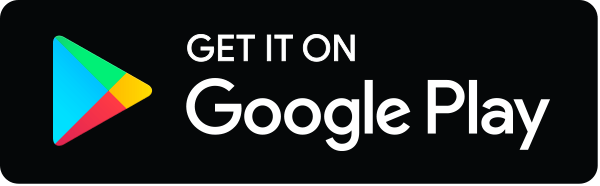