Fig. 6.1
Interaction of central nervous system (CNS) injury, CNS structure/function, and behavioral experience. While it is well known that after CNS injury, such as might occur in stroke or trauma, individuals spontaneously recover to varying degrees. Behavioral experience is a potent modulator of functional recovery and is often combined with various adjuvant or combinatorial treatments, such as cortical stimulation. However, it is not possible to completely isolate the independent effects of a treatment given the complex interplay of factors influencing functional outcome
Despite these promising approaches, clinical gains remain modest, and rehabilitative training protocols that are effective in populations with mild-to-moderate impairment are not feasible in more severely impaired individuals. By understanding the brain’s self-repair processes and the specific effects of therapeutic intervention on plasticity mechanisms, it may be possible to improve the trajectory of clinical recovery as well as to extend current treatment options to serve a broader population.
6.3 Response of Surviving Neurons to Cortical Injury
Following injury to the motor cortex, as often occurs in stroke , the affected tissue undergoes cell death, leading to a loss of function. This manifests itself as a reduction or loss of motor coordination and movement [18, 19]. Traditionally, neurologists have considered post-injury motor deficits to be the direct result of the loss of corticospinal fibers to the spinal cord motor neurons. But, in addition to the area of infarct, many other cortical areas are indirectly affected. The disruption of other corticofugal as well as corticocortical connections may be the basis for at least part of motor and sensorimotor deficits.
It is now well known that extensive reorganization occurs in neural circuits in the brain spared by the injury [20–24]. Research in nonhuman primates indicates that following a lesion to the primary motor cortex (M1), physiological reorganization can occur within the spared cortical areas, such as the area surrounding the lesion (peri-infarct) [23], or in other cortical areas, such as the premotor cortex [25]. These neurophysiological processes are assumed to be largely adaptive, perhaps contributing to behavioral recovery. A thorough understanding of the brain’s own self-repair processes may provide clues regarding the most appropriate approaches for restorative therapy.
Several factors provide an environment conducive for drastic physiological and anatomical changes following an infarct. Carmichael et al. examined gene expression of peri-infarct tissue following a lesion in the rat cerebral cortex and found up-regulation in genes related to growth proteins, synaptogenesis, dendritic sprouting, and angiogenic factors as well as down-regulation in genes related to growth inhibition [26]. Urban et al. did similar work in the rat targeting only neurons that projected into the ischemic core and found that many of the same factors were up- and down-regulated [27]. This would indicate that the neurons that survive the injury, but have connections with areas undergoing degeneration or necrosis, rapidly employ molecular mechanisms to promote neurite outgrowth . There is also an up-regulation of neurotrophic signals, such as BDNF, GDNF, and growth cone proteins, reduction in axonal growth-related inhibitory signals, as well as reduction in angiogenic factors and neuroprotective signals that may promote reinnervation of peri-infarct areas [24, 28].
One mechanism that may promote the targeting of the novel axonal projections could be the intrinsic patterning of neuronal activity. In the developing nervous system, it has been demonstrated that spontaneous neural activity is critical for long-distance targeting in the visual cortex [29, 30]. Further, this research shows that during development, an increase in the relative amount of activity in the retina drives expansion of development into the lateral geniculate nucleus [30]. Following an ischemic lesion in the adult rat, Carmichael found low-level waves of electrical activity at one and three days post lesion [31]. This activity was critical for sprouting, as treatment with TTX during this time extinguished the effect. It is possible that underlying neuronal activity is essential for long-range cortical sprouting.
During development, there are many chemical signaling molecules that induce and guide growth of axons in the cerebral cortex [32]. Evidence suggests that while chemical cues may guide growth, electrical activity can also serve as a guidance signal and is likely to be responsible for tuning and refining connections [33]. It appears that both chemical and electrical cues guide directional growth and promote axonal sprouting, especially in developing neural tissue [34–36]. There is, however, little empirical evidence to suggest that the normal adult mammalian neocortex can spontaneously form long-range anatomical connections in vivo. In the normal tissue, there are factors that limit the spontaneous outgrowth of axonal processes, such as the neurite outgrowth inhibitory protein, NOGO, and its receptors [33]. Most anatomical changes that occur are at the local level and include increased synaptic density, dendritic spine expansion, proliferation of neurotransmitter receptors, and dendritic arborization [37, 38]. These changes result from normal learning and adaptation. The plastic nature of the brain allows reorganization on a microscale that leads to global changes in behavior and functioning [39, 40].
The injured brain is capable of drastic neuroanatomical changes. This has been demonstrated in both squirrel monkeys and rats. In nonhuman primates, the ventral premotor cortex (PMv) normally sends dense projections to M1. Dancause et al. found that following a cortical lesion to M1 in squirrel monkeys, neurons projecting from PMv bypassed the injured M1, sending axons around the central sulcus to innervate areas 1 and 2 of the primary somatosensory cortex (S1), an area virtually devoid of these connections in normal, intact brains [20] (Fig. 6.2). In the rat, Napieralski found novel sprouting from the contralesional cortex to the ipsilesional cortex in animals with a unilateral sensorimotor lesion [22]. These two studies demonstrate that axonal growth over large distances is possible following a cortical lesion. Thus, the injured brain can no longer be considered as a normal brain with a destroyed module. The injured brain is extensively rewired, both from an anatomical and neurophysiological perspective. Yet, many of the rules that guide neuroanatomical sprouting and neurophysiological reorganization after injury, and the ultimate constraints on the system from the standpoint of timing and extent, are still largely unknown.
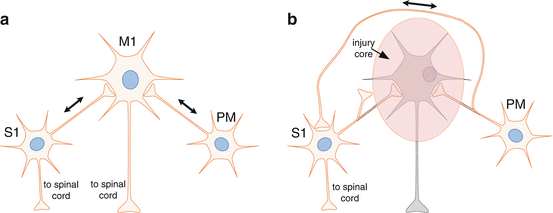
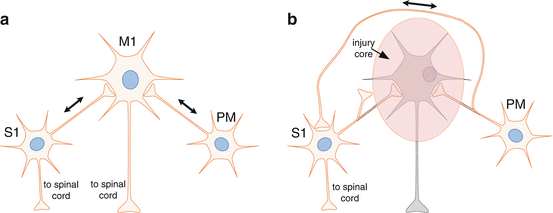
Fig. 6.2
The brain’s self-repair process. Injury to the CNS triggers a cascade of events that result in the expression of many growth-promoting as well as growth-inhibiting genes. The post-injury growth program has many similarities to that of early brain development. The post-injury growth phase lasts several weeks and can result in completely new pathways being formed. For example, after an injury to the primary motor cortex (M1), the spared premotor cortex (PM) sprouts axons that course around the injury to terminate in novel locations, such as the somatosensory cortex’s (S1) (a) normal connection patterns in the cerebral cortex. Single neurons are illustrated to represent ensembles of neurons in each cortical area. (b) Spontaneous rewiring of connection patterns after a focal injury in M1. Dotted outlines indicate degenerated neurites. It is possible that such rewiring represents an attempt by the nervous system to reintegrate somatosensory and motor information [20]
6.4 Functional Integration Between Cortical Areas and Its Disruption Following Injury
The brain is composed of millions of neuronal connections spanning hundreds of distinct anatomical and physiological areas. For much of neuroscience history, brain regions were described based on their appearance under neuroanatomical stains, electrophysiological input and output properties, and neuroanatomical tracing studies. As such, specific regions of the cerebral cortex and their properties were generally described as individual units or modules with cursory attention to their interaction with the rest of the brain. While this research has been critical for understanding the architecture and input/output properties of various cortical areas, it has given us a relatively compartmentalized view of the brain function.
Diverse studies examining the extensive anatomical connectivity as well as fMRI resting-state connectivity have made clear that the interplay of communication among brain regions is critical for overall function [41–46]. Something as seemingly simple as performing a reach with the arm requires communication from many different cortical and subcortical regions even before corticospinal output from M1 occurs, and throughout the movement, feedback communication is constantly correcting for movement errors [47]. For these basic movements, the importance of communication becomes apparent when observing the effects of injury to any of the areas that are involved in the function [19, 48–51].
Recently, there has been a trend to investigate the effects of brain damage on the communication between the areas spared from injury. The loss of communication both to and from the motor cortex, not just the loss of the motor cortical tissue and its spinal cord projections, could be the cause of many of the long-term deficits seen from acquired brain injuries. Corbetta and others have used fMRI to examine the resting-state connectivity between functional areas in both normal individuals and those with damage due to stroke or TBI [41, 42, 44, 46]. Their results indicate a substantial change in the activity within and between functional areas. This change in activity could be the result of beneficial neural plasticity or could indicate maladaptive plasticity that impairs recovery from injury.
Additionally, there is evidence that one of the critical components of skilled motor movements is the communication between the somatosensory cortex and motor cortex. It is necessary to have feedback on both joint position and tactile sensation in order to perform skilled movements. While M1 is usually considered as a motor output structure, it receives somatosensory input from S1 that is segregated based on submodality. Cutaneous afferents project largely to the posterior part of M1, while proprioceptive afferents project to the anterior part of M1 [52]. Functional deficits after lesions in M1 reflect the specific somatosensory submodality that is represented at the site of the lesion. After a lesion restricted to posterior M1, monkeys can retrieve small food pellets, but they look into their hand, as if they did not know that the pellet was there. This is similar to a sensory agnosia in humans. After a lesion restricted to anterior M1, monkeys make trajectory errors, overshooting the target [53]. Monkeys compensate, as do humans, by using visual guidance to aid in motor control. After injury, other motor cortical areas may have the ability to play a vicarious role in recovery, but they may not share corticocortical connections with the somatosensory cortex [54, 55]. Based on a number of studies, it is clear that the observed deficit from an injury is a combination of impaired function of the tissue directly affected as well as the lack or miscommunication to and from spared cortical areas [45, 53, 54] (Fig. 6.3).
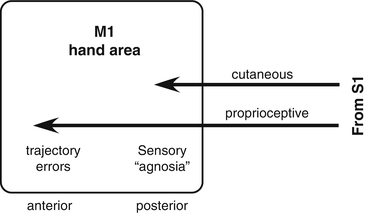
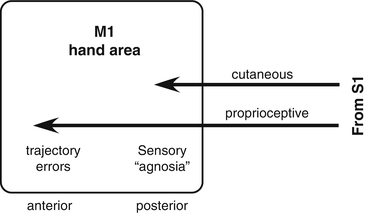
Fig. 6.3
Sensorimotor integration in the primary motor cortex (M1). While the motor cortex is typically considered an output structure, due to its tight coupling with motor neurons in the spinal cord, it is also an important integrator of somatosensory information and motor programs received from premotor areas. Normal, skilled motor function requires this sensorimotor integration. When lesions occur in the posterior M1, the site of termination of cutaneous afferents, a sensory agnosia occurs. When lesions occur in the anterior M1, the site of termination of proprioceptive afferents, trajectory errors occur [53]
Thus, the disrupted communication between somatosensory input and motor output may underlie at least some of the deficits seen after M1 injury. Perhaps this is why after M1 injury, the premotor cortex sends de novo projections to S1, and reciprocal projections from S1 neurons likewise project back to the premotor cortex [20]. The brain’s intrinsic self-repair processes may be attempting to restore communication between sensory inputs and motor outputs. In the days and weeks following a cortical injury, spared cortical areas alter the pattern of their projections, either innervating novel areas or strengthening existing connections that existed prior to the lesion [20, 22, 31]. This is thought to be the result of altered firing patterns (which are critical for novel axonal sprouting), as well as the release of various growth factors following an injury [26, 27, 31]. While it is unclear what role this novel sprouting plays in functional recovery, this attempt to reestablish communication between spared areas may be a significant driving factor in recovery. If these intrinsic processes can be amplified and controlled via neuroprosthetic interfaces, they may be able to have significant impact on recovery after brain lesions.
6.5 Current Neuroprosthetic Strategies
Neuroprostheses cover a wide variety of technologies that allow manipulation of neural activity through electrical or mechanical means. For purposes of treating neurological conditions, neuroprostheses generally, they fall into two categories: (1) neuromodulation of CNS activity by electrical or magnetic stimulation and (2) decoding the rate or pattern of neural activity to control external devices such as computer input devices or robotic limbs. The first category contains well-known clinical devices, such as cochlear implants, deep brain stimulators, and vagal nerve stimulators (Fig. 6.4a). The second category contains many of the experimental and preclinical devices that attempt to restore communication and motor function to those with conditions such as spinal cord injury, paralysis, and locked-in syndrome. The term “brain–computer interface ” is most often associated with this type of neuroprosthetic device.
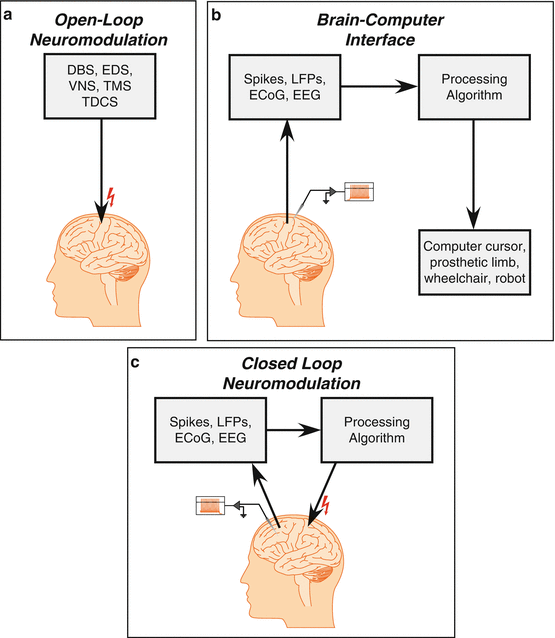
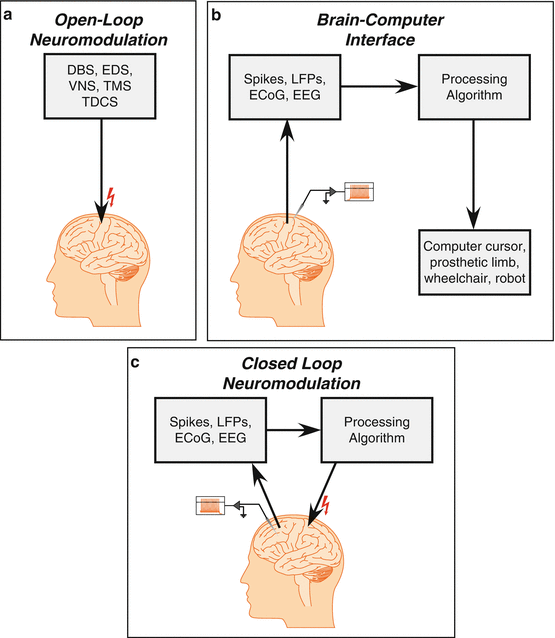
Fig. 6.4
Neuroprosthetic strategies. Current neuroprostheses fall under one of the three modes of operation. (a) Open-loop neuromodulation is the most common mode and includes such relatively common clinical devices as cochlear implants, deep brain stimulators, and vagal nerve stimulators. (b) The so-called brain–computer interface (sometimes “brain–machine interface”) while still investigational, utilizes neural signals from intact regions of the CNS to define output commands to control external devices, such as computer cursors or robots. Brain–computer interfaces have been successfully employed in small numbers of paralyzed individuals and hold great promise for clinical application in the near future. (c) Closed-loop neuromodulation, sometimes called a “brain–machine–brain interface,” is just beginning to be tested using implantable systems in preclinical models and, to date, noninvasive systems in clinical populations. Closed-loop approaches have the potential to potentiate the functional connectivity of brain regions, potentially restoring function in a wide variety of clinical applications
An initial route of investigation into promoting recovery after M1 injury was to use electrical stimulation to modulate activity in spared motor areas. Because a change in firing characteristics exists in the adjacent areas surrounding the lesion due to suppressed neural activity, several studies have attempted to use epidural stimulation in the peri-infarct area to promote behavioral recovery. By applying subthreshold levels of current, in combination with rehabilitative training, there was a reduction in the resting potential needed to drive action potentials in the spared cortical areas. This makes these neurons more likely to fire, and this activity may lead to potentiation that promotes recovery. Epidural or subdural stimulation in both rodent and primates following injury has been shown to elicit small, but significant, gains in motor performance. Adkins et al. found that following an ischemic lesion to M1 of the rodent, stimulation currents that were subthreshold for producing movements could promote a small but significant increase in retrieval performance [56, 57]. Plautz et al. showed similar results in nonhuman primates [58]. Defined end points of a clinical trial using this approach failed to find significant improvements in motor outcomes, but indicated that when used in areas that are still responsive following a cortical infarct stimulation treatment was beneficial [59].
While there has been little work specifically attempting to use the activity of the spared cortical areas to assist with rehabilitation of movement, a substantial amount of related research has significant therapeutic potential. In the early 1980s, Georgopoulos showed that a majority of neurons recorded in M1 responded preferentially to a particular direction [60, 61]. This led to the idea that directionality of motor movements could be decoded by recording from neurons and that it was possible to predict the movement trajectory based on that activity [62]. With the introduction of multichannel microelectrodes , a jump was made from being a one-dimensional system (essentially the firing rate can be coded to a binary state or to linear motion) to recording from multiple units that allows for many degrees of freedom. This is extremely beneficial when trying to drive a prosthetic limb with kinematic patterns that approximate human movement patterns or control complex computer user interfaces.
The increase in channels required a corresponding increase in computing power. As more channels were used for algorithms for determining output, it was necessary to record the output of each channel and then execute the decoding algorithm and generate an output virtually in real time. As the decoding strategies improved over time, the algorithms used for decoding became more complex and the predictors for the movement were refined. This result has been an array of successful BCIs ranging from control of robotic arms to computer cursors and speech [63–66].
While both strategies show promise in restoring function, there are substantial drawbacks with each. Using cortical stimulation may promote minimal recovery of function in a selected population (mild-to-moderately impaired) when coupled with rehabilitation strategies, but the stimulation is nonspecific, and it is unclear whether the stimulation itself promotes the recovery or if the stimulation creates a more malleable environment for plasticity to occur. Using the output of spared neurons to control external signals may be a viable solution for certain conditions, but they currently require enormous support systems for both the technology and the patient. Further, while these systems have substantial clinical potential, they largely enable compensatory strategies, providing the patient with the ability to interact with the environment but using external devices (computer cursors, robots, etc.). Ultimately, BCIs could potentially be used for controlling intraspinal stimulation or even muscles directly; there are currently significant technological and biological hurdles. Despite their current limitations, the value of enabling a paralyzed and/or locked-in individual to interact with their environment cannot be minimized, and further advances are likely to make such systems more feasible in the near future.
6.6 Hebbian Plasticity and the “Neurochip ”: A Prelude to Closed-Loop Neuroprosthetics
In The Organization of Behavior, Donald Hebb proposed a rule that governs the relative strength of interactions between neurons, stating: “When an axon of cell A is near enough to excite cell B and repeatedly or persistently takes part in firing it, some growth process or metabolic change takes place in one or both cells such that A’s efficiency, as one of the cells firing B, is increased” [67]. The validity of this rule has been shown in many examples, particularly stemming from research into learning and long-term potentiation (LTP). This idea that “cells that fire together, wire together” is critical for the plastic nature of the brain. The ability to adapt relative strengths of firing is what drives learning, both in normal conditions, but also those presented following an injury. While Hebb’s rule is typically applied to local circuits, likely mediated by simple changes in synaptic strength, it is possible that the pairing of activity can drive much more extensive physiological and perhaps anatomical rewiring.
This postulate has gained strength primarily through simple organisms and hippocampal slice studies purportedly examining memory formation [68]. There have also been cellular demonstrations in vivo; within the motor cortex of the cat, paired conditioning pulses could evoke synaptic facilitation after fewer than 90 trials when applied within 100 ms of the initial pulse [69]. Neurons in the visual cortex could shift orientation selectivity toward the initial population following synchronous pairing, indicating a shift in output properties of these cells was possible and timing-dependent [70]. Additionally, LTP in the basal ganglia could be induced by stimulating the ipsilateral orofacial area of the rat [71]. These studies examined the effects of individual neurons within an area while manipulating both the activity in the initial population and the stimulation of the subsequent area in anesthetized animals.
Most importantly for the present model, Jackson and colleagues used activity-dependent stimulation provided by a device called the “Neurochip ,” to alter output properties of pyramidal neurons in M1 of an intact, behaving macaque monkey. In this study, action potentials recorded from one cortical module were used to drive microstimulation of another cortical module that was located 1–2 mm away. Following only 2 days of entrainment, the muscle responses evoked with test stimuli at the recording site closely resembled the responses at the stimulation site and remained stable for 1 week. These results were explained as deriving from the strengthening of intracortical connections within the motor cortex [72]. These studies of activity-dependent stimulation demonstrate that functional relationships can be built in the intact adult nervous system by artificially driving temporal sequences of inputs and outputs of cortical modules, at least over short distances. Given the small distance between the electrode sites (1–2 mm), strengthening of intrinsic horizontal fibers, connecting various parts of the motor cortex, via LTP-like mechanisms can indeed account for such changes even in the absence of anatomical reorganization. In related studies in awake, behaving rats, Rebesco and Miller found that spike-triggered stimulation in the sensorimotor forelimb area of the cerebral cortex induced increases in statistically inferred functional connectivity (IFC) [73]. The IFC estimate was based on Bayesian approaches used in other studies. After 2 days of spike-triggered stimulation, the IFC increased, but only with specific spike-triggered delays. When a 5 ms delay was used, IFC changes were robust; when a 500 ms delay was used, no changes in IFC occurred. Most importantly for applications to neurological recovery, the spike-triggered stimulus paradigm resulted in a reduction in the detection threshold for an ICMS pulse. Thus, targeted spike-triggered stimulation can result in behavioral changes in the animal.
The fact that very long-range corticocortical and corticostriatal connectivity is spontaneously altered after injury, and that synchronous oscillatory discharge is necessary for the new connections to be formed, combined with the demonstration that new functional relationships can be formed in intact cortex over short distances sets the stage for implantable device technology to orchestrate long-range connections after neuronal injury. There is evidence to suggest that while chemical cues may guide growth during development and after injury, electrical activity can also serve as a guidance signal and is likely to be responsible for tuning and refining connections [33]. In tissue slices, some of these guidance cues can be used to induce directional growth and promote axonal sprouting. If paired activity drives strengthening of these anatomical connections and the conditions in the injured cortex are amenable to axonal sprouting, it may be possible to guide axonal growth through activity-dependent stimulation. This could lead to targeted repair and plasticity of the remaining cortex and potentially a new method of promoting recovery following injury.
6.7 Differential Mechanisms Underlying Plasticity in Open-Loop vs. Closed-Loop Stimulation Approaches
If closed-loop approaches are to be considered, it is important to understand what differential effects open-loop vs. closed-loop stimulation might have on plasticity of the injured brain. Open-loop stimulation has inherent advantages in that, theoretically, noninvasive stimulation may be possible. To implement the closed-loop technology afforded by the Neurochip, in which action potentials are used to trigger remote stimulation, it is currently necessary to implant penetrating microelectrodes into the cortical tissue. While various schemes using electrocorticography or even electroencephalography have been proposed as control signals to stimulate using noninvasive methods [74], these approaches lack the temporal resolution of neuronal action potentials . It is possible that millisecond timing will be required to optimally utilize the brain’s intrinsic synaptic potentiation mechanisms.
Evidence to support specific mechanisms underlying the effects of open-loop, cortical electrical stimulation on recovery is still largely correlative, but includes motor map reorganization, increased dendritic length and spine density, increased cell proliferation and cell migration in the subventricular zone, receptor subunit expression, activation of antiapoptotic cascades, increased c-fos expression, increased neurotrophic factors, enhanced angiogenesis, and proliferation of inflammatory cells ([57, 58, 75–81]. Thus, electrical stimulation, whether delivered in open-loop or closed-loop mode, has the potential to promote proliferative events in various cellular elements in the brain.
In addition, various open-loop stimulation protocols are known to produce alterations in synaptic efficacy. LTP is likely to be expressed in both excitatory and inhibitory synapses throughout the mammalian brain [82]. While many experimental protocols have been developed to optimize LTP in various systems, the sign and magnitude of synaptic potentiation are heavily dependent upon the frequency and pattern of stimulation, with LTP induced with high-frequency bursts and long-term depression (LTD) induced at low frequencies [83]. Such high-frequency bursting patterns, known as theta-burst stimulation , are often used to optimize generation of LTP, especially in the cerebral cortex of awake animals, where LTP is notoriously difficult to produce [84]. In a study in the cortex of freely moving rats, theta-burst stimulation using parameters similar to those used to evoke LTP in hippocampus was used. While LTP was generated, the effects required at least 5 days to develop and plateaued at about 15 days [85]. These studies suggest that open-loop stimulation protocols can induce plasticity in synapses of the cerebral cortex, but the precise parameters do not translate directly from the results of reduced preparations (e.g., slice preparations).
Theta-burst timing protocols vary considerably depending upon the particular model system. However, a recent study in a mouse brain slice preparation in the dorsal striatum suggests that the optimal theta-burst patterns are those that best match intrinsic neural activity patterns [86]. An intra-burst frequency of 50 Hz produced stronger LTP than 100 Hz. Further, “burstiness” was critical to inducing LTP in the dorsal striatum. Simply reducing the inter-burst pause from 35 to 20 ms eliminated the induction of LTP.
While LTP processes may be optimal at slightly different intra-burst and inter-burst frequencies in different neuronal populations (hippocampus vs. dorsal striatum vs. cerebral cortex), these studies suggest that LTP phenomena are optimized when the stimulation parameters closely match intrinsic firing patterns. Existing data suggest that there is both a period of hyperexcitability and increased firing rates in the spared tissue up to 2 weeks post-injury [30, 87]. This pattern of activity is likely to be critical for the formation of novel connections. We propose that intrinsic stimulation patterns optimally drive synaptic potentiation in corticocortical pathways and can lead to long–lasting functional connectivity between distant cortical areas.
Thus, open-loop stimulation and activity-dependent stimulation both may alter neuronal structure and function, but by different mechanisms. Electrical stimulation, in general, is likely to modulate neuronal growth processes, leading to some degree of adaptive plasticity that could account for behavioral improvement observed in the many studies demonstrating the effects of invasive and noninvasive stimulation after cortical injury. These processes are likely to be modulated in both open-loop and closed-loop systems. In closed-loop, activity-dependent stimulation, however, the intrinsic firing pattern may drive synaptic potentiation in a manner similar to that observed in theta-burst protocols.
6.8 Future Applications of Closed-Loop Neuroprostheses for Treating Neurological Disorders
A closed-loop neuroprosthetic microdevice applying activity-dependent stimulation across distant cortical areas is a vastly different approach to brain repair than has been achieved to date. Neuroprosthetic approaches employing closed-loop stimulation in the brain are still uncommon. However, closed-loop approaches are already being tested for epilepsy, and an expanded role for closed-loop systems for DBS in Parkinson’s disease is now being considered [88–90]. Further, various closed-loop approaches are under development in animal models of spinal cord injury [91, 92]. Other investigators have proposed a closed-loop approach for the development of a cognitive prosthesis that has shown promise in animal models [93]. Other potential clinical applications based on the current model include stroke, focal TBI, and surgical resections. Finally, a variety of neurological syndromes that are thought to be related to disruption of cortical communication may be amenable to activity-dependent stimulation. In the 1960s, Norman Geschwind identified several disorders collectively called “disconnection syndromes ,” revolutionizing the field of behavioral neurology [94]. The consideration of closed-loop approaches to repair cortical disconnection syndromes may open up treatment options for a variety of conditions in which neural communication is disrupted, whether due to disease, injury, or idiopathic causes.
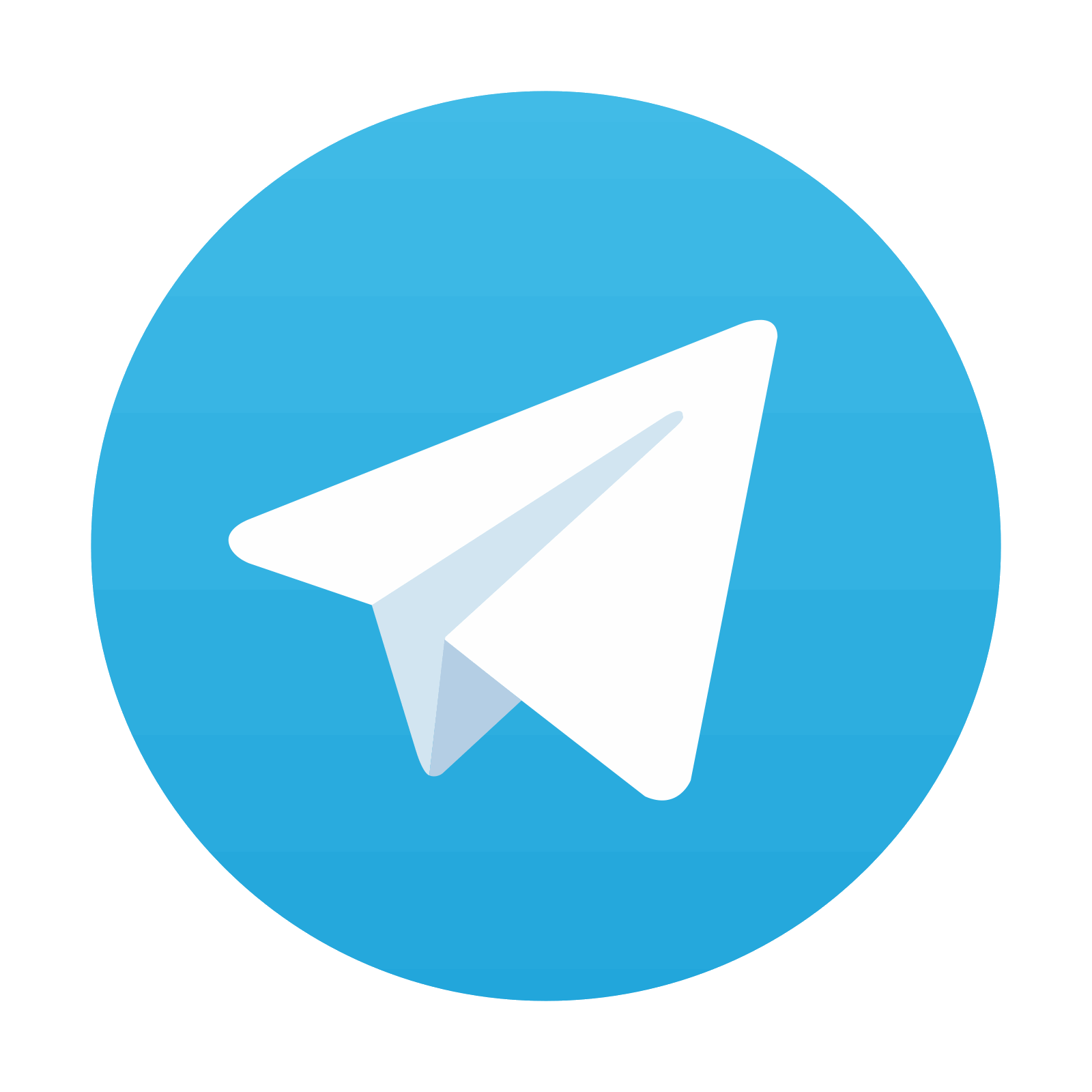
Stay updated, free articles. Join our Telegram channel
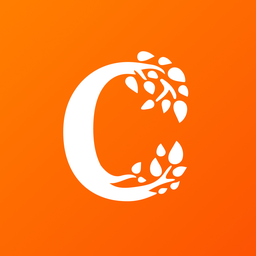
Full access? Get Clinical Tree
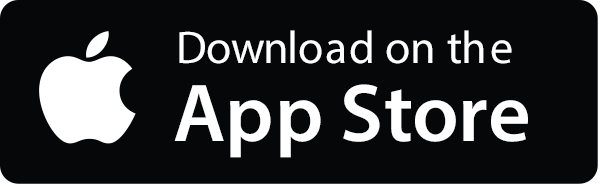
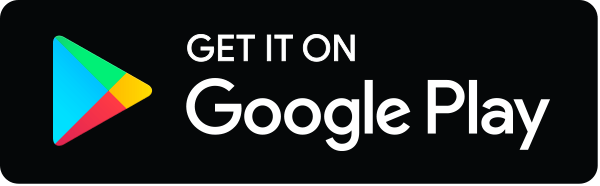