Figure 12.1 Experimental design of the selective working memory task. Tasks differed in the instructions given at the beginning of each run and in the response requirements. Participants were instructed to (1) Remember faces and ignore scenes, (2) Remember scenes and ignore faces, and (3) Passively view both faces and scenes – with no attempt to remember or evaluate them. In the memory trials response period, a face or scene stimulus was presented (depending upon the condition), and participants were required to report with a button press whether the stimulus matched one of the previously presented stimuli. During the response period of the Passive view task, an arrow was presented and participants were required to make a button press indicating the direction of the arrow. (Adapted from Gazzaley et al. (2005a) J Cogn Neurosci, 17(3), 507–517.)
faces and scenes without attempting to remember them. In each task, the period in which the cue stimuli are presented is balanced for bottom-up visual information, thus allowing us to probe the influence of goal-directed behavior on neural activity (top-down modulation). In the two memory tasks, the encoding of the task-relevant stimuli requires selective attention and thus permits the dissociation of physiological measures of enhancement and suppression relative to the passive baseline. For example, measures of neural activity above passive baseline reflect enhancement, and activity below baseline is suppression. Also in the memory tasks, after a 9 s delay, the participants are tested on their ability to recognize a probe stimulus as being one of the task-relevant cues, yielding a behavioral measure of WM performance. In addition, a post-experiment surprise recognition memory enables us to evaluate incidental long-term memory of the stimuli.
The experiments we performed using this paradigm employed both event-related functional MRI (fMRI) and electroencephalography (EEG) on counterbalanced sessions to record correlates of neural activity while the participants performed the task. This allowed us capitalize on the high spatial resolution achievable with the fMRI Blood Oxygen Level Dependent (BOLD) signal and the high temporal resolution attained when recording electrical activity with EEG. Although both measures are thought to reflect cortical activity driven by local cortical processing and the summation of postsynaptic potentials on synchronously active, large ensembles of neurons (Chawla, Lumer and Friston 1999; Logothetis, Pauls, Augath et al. 2001; Silva 1991), changes in BOLD signal can be localized to cortical regions separated by millimeters and EEG can resolve activity changes in the millisecond range. Thus these techniques offer complementary but unique information to study the modulation of activity at the neuronal population level.
Our original research focus was to identify neural measures of both the enhancement and suppression of neural activity associated with task-relevant and task-irrelevant information, respectively. Inherent to theories of top-down modulation is the concept that neural activity is modulated relative to a level of activity generated when a stimulus is passively viewed and no goal-directed decisions are performed, i.e. its bottom-up, perceptual influence. Neural activity in (p.200) response to viewing a stimulus may be differentially enhanced or suppressed relative to this level of activity if it is respectively attended or ignored. Despite this logic, modulation relative to a stimulus-present, neutral baseline has rarely been evaluated and comparisons are usually made between attend and ignore tasks or relative to a resting baseline without visual stimulation (Eimer 2000; Holmes, Vuilleumier and Eimer 2003; O’Craven, Downing and Kanwisher 1999; Pinsk, Doniger and Kastner 2004; Rees, Frith and Lavie 1997; Vuilleumier, Armony, Driver et al. 2001; Wojciulik, Kanwisher and Driver 1998). Without establishing a perceptual baseline level of activity, it is not possible to interpret top-down influences as representing enhancement or suppression. While modulation both above and below a perceptual baseline has not yet been reported with neuroimaging data, the presence of enhancement and suppression has been suggested in EEG studies of spatial attention documenting a decreased amplitude of the P1 component for ignored locations and increased amplitude of the N1 component for attended locations, both relative to a baseline obtained with ‘neutral’ trials when attention was unfocused or broadly focused (Luck and Hillyard 1995; Luck et al. 1994). The passive viewing task utilized in our experiment established a perceptual, bottom-up baseline from which activity in the remember tasks could be compared.
We chose to focus our first study on activity measures of enhancement and suppression obtained from visual association cortex of young healthy participants. For fMRI, we used an independent functional localizer to identify both stimulus-selective face regions and scene regions in the fusiform gyrus and the parahippocampal/lingual gyrus, respectively. For the purpose of this chapter, we will focus on the fMRI data from the left scene-selective region since it yielded the most robust measures of top-down modulation. For EEG, we utilized a face-selective event-related potential (ERP), the N170, a component localized to posterior occipital electrodes and reflecting visual association cortex activity with face specificity (Bentin, Allison, Puce et al. 1996). Our fMRI and EEG data revealed top-down modulation of both activity magnitude and processing speed occur above and below a perceptual baseline depending on task instruction (Figure 12.2). Modulation of the processing speed as reflected by a shift in the latency of the N170 was a novel finding that revealed another aspect of top-down modulation. It suggested that in addition to modifying activity magnitude, top-down influences can modulate the time-course of neural activity, as reflected by a shorter time to reach maximal synchronized neural activity (Silva 1991). It has been proposed that amplification of activity magnitude improves signal/noise ratio, allowing more information to be extracted from relevant stimuli (Hillyard, Vogel and Luck 1998). Likewise, faster processing speed reflects an augmentation in the efficiency of neural processing, further facilitating information extraction.
It is well documented that the nervous system utilizes interleaved inhibitory and excitatory mechanisms throughout the neuroaxis (e.g., spinal reflexes, cerebellar outputs and basal ganglia movement control networks). It is thus not surprising that top-down modulation would utilize both enhancement and suppression to control the impact of sensory information on neural activity, providing a powerful contrast for sculpting these neural processes (Knight, Staines, Swick et al. Chao 1999; Shimamura 1997). Thus, by generating contrast via enhancing and suppressing activity magnitude and processing speed, top-down signals bias the likelihood of successful representation of relevant information in a competitive system.
A unique aspect of this study was that the relevant and irrelevant stimuli were presented sequentially. Most selective attention studies that have assessed activity modulation have used tasks in which multiple stimuli were presented simultaneously(O’Craven, Downing and Kanwisher 1999; Vuilleumier, Armony, Driver et al. 2001; Wojciulik, Kanwisher and Driver 1998), and so modulation was considered to be driven by competition for limited perceptual processing resources. In contrast, our findings reveal that modulation of activity magnitude can occur based (p.201)

Figure 12.2 Activity data for Remember and Ignoreconditions: fMRI and ERP. (a) Group data: Average beta values in the scene selective area revealing greater activity in Remember scenes vs Ignore scenes condition. (b) A representative subject demonstrating the BOLD signal level within the masked scene selective in Remember scenes vs Ignore scenes condition. (c) Group data: Average peak latency for the right N170 in PO8 electrode revealing earlier latency for Remember faces vs Ignore faces. (d) Grand-averaged waveforms of the time-locked ERPs to face stimuli revealing earlier latency for Remember faces vs Ignore faces. Error bars indicate standard error of the mean. (Adapted from Gazzaley et al. (2005a) J Cogn Neurosci, 17(3), 507–517.) See also color plate 5.
Enhancement and suppression: dissociable processes?
Using the selective WM paradigm we developed, we are able to generate distinct measures of top-down enhancement and suppression by calculating the fMRI BOLD magnitude and ERP component amplitude or latency difference between the remember condition and passive view (enhancement), or between passive view and the ignore condition (suppression). An important question that emerged was whether or not these two processes are mechanistically dissociable? If they have independent control processes, either anatomically or neurochemically, then they might be differentially affected by aging or disease. Our first attempt at addressing this was to (p.202) study top-down modulation using this paradigm in a population of healthy older participants (Gazzaley, Cooney, Rissman et al. 2005b). In this population, we predicted that we would see a shift in the balance between enhancement and suppression. It is well-established that many aspects of cognition decline with normal aging (Craik and Salthouse 2000). However, behavioural evidence exploring the interaction between attention and WM in aging suggests that age-related WM impairments are associated with increased sensitivity to interference from task-irrelevant information (Hasher and Zacks 1988; May, Hasher and Kane 1999; West 1999). We hypothesized that the older individuals may have a selective deficit in their ability to suppress task-irrelevant information. Such a select deficit would provide evidence for dissociable mechanisms of enhancement and suppression.
In a recent publication, we compared the fMRI BOLD signal magnitude between the tasks within each group of younger (n = 17, 19–30 years of age) and older participants (n = 16, 60–77 years of age) (Figure 12.3). Direct comparisons of BOLD signal across age groups revealed a significantly greater signal magnitude within the scene-selective region in the older group than in the younger group in the Ignore scenes condition (p ‹0.005), while no age-related differences existed between the Remember scenes (p = 0.37) or Passive view conditions (p = 0.96). These comparisons reveal the presence of a selective age-related deficit in the suppression of task-irrelevant information. To further compare across age groups, we calculated three modulation indices: overall modulation index (Remember scenes – Ignore scenes), enhancement index (Remember scenes – Passive view) and suppression index (Passive view – Ignore scenes). The use of these indices enabled across-group comparisons to be performed without directly contrasting BOLD signal magnitude between populations that might have vascular responsivity differences

Figure 12.3 Relationship of suppression deficit and WM deficit. (a) and (c) Across-group comparisons of (a) Face WM accuracy (* p = 0.001) and (c) Suppression indices (* p‹ 0.005). (b) Subgroups of the six high performing and six low performing older individuals (*p‹10–5) on the Remember faces condition. (d) A significant suppression deficit is only present in the low performing older subgroup (*p‹0.05). Error bars indicate standard error of the mean. (Adapted from Gazzaley et al. (2005b) Nat Neurosci, 8(10), 1298–1300.)
Furthermore, we determined that only the subpopulation of older adults with a significant WM deficit on the task had a significant suppression deficit. This subpopulation also rated the scenes that were viewed during the Ignore scenes task as significantly more familiar than the younger participants rated them on the surprise post-experiment recognition test, revealing increased incidental long-term memory of distracting information and supporting our neural data that task-irrelevant scenes were not suppressed. This established the relationship between an age-related deficit in selective attention (specifically the suppression of task-irrelevant information), incidental long-term memory encoding and interference during the WM task. This finding, in addition to yielding important information about normal aging, revealed that enhancement and suppression, as defined by our measures, are dissociable processes.
To further explore the dissociation between the neural mechanisms of enhancement and suppression, we have begun experiments to manipulate the cognitive demands of the task and evaluate its effect on these measures. In one experiment, we are attempting to determine if having participants perform a nonverbal working memory task concurrently with the visual selective attention working memory task will differentially affect enhancement and suppression measures. At the beginning of each trial, participants were presented auditorily with six digits to memorize. On half of the trials the digit sequence was random (high load); on the other half the digit sequence was ‘1, 2, 3, 4, 5, 6’ (low load). After hearing the digits, the participants then performed the face/scene WM paradigm as previously described. Preliminary results revealed that the high digit load did not alter the participants’ ability to enhance activity levels in the scene-selective region during the Remember scenes task, but did result in increased BOLD signal associated with the irrelevant scenes in the Ignore scenes task (Rissman, Gazzaley and D’Esposito 2005). The fact that increased WM load in younger adults produced a selective suppression deficit similar to that seen in older adults suggests that age-related changes in top-down modulation may, in part, result from decreased WM resources with age.
These data and the findings on older participants converge to suggest that enhancement and suppression processes are dissociable. In these studies, only the measure of suppression was influenced by aging and increasing memory load, while the enhancement index was unchanged. This suggests that they are differentially regulated and thus may have different anatomical and neurotransmitter control systems.
Where is the top?
Identification of distinct and dissociable measures of top-down enhancement and suppression in visual association cortex raises the important question of which brain regions drive top-down modulation? It has been suggested that the top-down modulation is not an intrinsic property of sensory cortices, but rather is achieved by intricate neural connections subserving dynamic interactions between brain regions, or neural networks. Tract-tracing studies in monkeys reveal an intricate anatomic network of reciprocal corticocortical connections between regions in the prefrontal cortex (PFC) and parietal cortex and the visual association cortex (Cavada and Goldman-Rakic 1989; Petrides and Pandya 1999, 2002; Ungerleider, Gaffan and Pelak 1989; Webster, Bachevalier and Ungerleider 1994). Several of these pathways have also been described in humans with post-mortem dissection (Heimer 1983) and more recently with in vivo diffusion tensor magnetic resonance imaging (Makris et al. 2004). These anatomically defined networks (p.204) establish the structural basis by which the PFC may exert control over diverse cognitive processes, and there is also accumulating neurophysiological evidence of PFC networks and their role in control processes. Neuronal recordings and neuroimaging data have revealed that top-down modulation of visual processing involves simultaneous activation of these regions (Corbetta 1998; D’Esposito et al. 1998; E. K. Miller, Li, and Desimone 1993; Moran and Desimone 1985; Ungerleider, Courtney and Haxby 1998). In addition, we observe increased BOLD signal in prefrontal and parietal regions in the memory tasks of our paradigm relative to the passive view task, suggesting a role of these regions as a ‘top’ in the visual association cortex activity modulation. It is important to note that the majority of these studies, including our own, reveal indirect evidence of functional interaction between these areas based on univariate statistics, which measure the activity in brain regions independently of other regions.
A noninvasive approach to evaluate interactions between regions with preserved structure and function is via multivariate analysis, a statistical method that used to generate maps of functional connectivity between brain regions during different cognitive processes (Buchel and Friston 2000; Friston, Phillips, Chawla et al. 2000; K. J. Friston, Frith, Liddle et al. 1993; Lin et al. 2003; McIntosh 1998; Penny, Stephan, Mechelli et al. 2004; Sun, Miller and D’Esposito 2004). Multivariate analyses generate functional and effective connectivity maps of interacting brain regions by measuring the activity relationship between anatomically connected regions and the cognitive processes being performed. We have recently developed a new multivariate method, designed specifically to characterize functional connectivity in an event-related fMRI dataset and measure interregional correlations during the individual stages of a multistage cognitive task (Rissman, Gazzaley and D’Esposito 2004). The method, beta series correlations, employs a standard general linear model (GLM) approach as do most univariate analyses for estimating stage-specific activity (Friston et al. 1995), but adapts the model such that distinct parameter estimates are computed for each trial and then used as the dependent data in a correlation analysis. Another important aspect of the technique is the use of a ‘seed’ region to explore the network correlated with a selected region and thus associated with a particular cognitive process. We have recently validated this method as a suitable measure of functional connectivity (Rissman, Gazzaley and D’Esposito 2004) and characterized the brain regions that revealed significant correlation with a visual association cortex seed during the maintenance period of a WM task (Gazzaley, Rissman and Desposito 2004). This maintenance network included the dorsolateral and ventrolateral PFC, premotor cortex, intraparietal sulcus, caudate nucleus, thalamus, hippocampus and occipitotemporal regions. These findings support the notion that the coordinated functional interaction between nodes of a widely distributed network underlies the active maintenance of a perceptual representation.
We are now in the process of performing a comparable functional connectivity analysis on the encoding phase of the selective working memory task we have described. Preliminary evidence has revealed regions of robust functional connectivity between the PFC and visual association cortex seeds during the encoding period, further supporting the role of the prefrontal cortex as a control region. However, there are only minimal differences between the connectivity patterns in the PFC associated with enhancement versus suppression. Using this dataset and the beta series correlation analysis, we have further revealed that functional connectivity between a visual association cortex seed and both the hippocampus and a region of the PFC, the inferior frontal gyrus, correlated with incidental long-term memory recognition when evaluated across subjects: thus establishing the utility of connectivity measures to predict cognitive performance (Siebert, Gazzaley, Rutman et al. 2005).
Although there is accumulating evidence that the PFC mediates its influence over diverse mental processes by modulating the magnitude of neural activity in distant brain regions via the (p.205)long-range projections, the majority of the evidence, including most multivariate analyses, are correlational. These studies only support the engagement of PFC in these cognitive processes and do not establish the casual relationship between PFC and control via top-down modulation. An optimal experimental design to directly assess the mechanism of PFC control involves the disruption of PFC afferents and physiological recordings of distant brain regions while the subject is engaged in a control task. There have been several studies that have implemented such a lesion-physiology design on experimental animals and humans. These studies support the conclusion that top-down modulation, utilizing both enhancement and suppression, is a mechanism of PFC control over diverse cognitive processes.
Research on experimental animals provided the first direct electophysiological evidence of a PFC role in modulating activity in sensory cortices. It was observed that cooling the PFC in cats results in increased amplitudes of evoked electrophysiological responses recorded from the primary cortex for all sensory modalities (Skinner and Yingling 1977). Conversely, stimulation of specific regions of the thalamus that surround the sensory relay thalamic nuclei (i.e. nucleus reticularis thalami) results in modality specific suppression of activity in primary sensory (Yingling and Skinner 1977). Thus, these findings suggest the presence of an inhibitory pathway from PFC that regulates the flow of sensory information via thalamic relay nuclei. This prefrontal-thalamic inhibitory system provides a mechanism for modality specific suppression of irrelevant inputs at an early stage of sensory processing.
In nonhuman primates, PFC mediated top-down modulation during a WM task was studied by coupling single-cell recordings and cortical cooling in monkeys (Fuster, Bauer and Jervey 1985). This experiment revealed that PFC cooling results in both augmentation and diminution of spontaneous and task-specific activity in inferotemporal neurons during the encoding (stimulus-present modulation) and delay period (stimulus-absent modulation) of a visual delayed-response task, suggesting the presence of both enhancing and suppressive PFC influences. Furthermore, cooling was accompanied by WM performance deficits, thus establishing a link between PFC-mediated top-down modulation and cognition. These findings have been complemented by the elegant callosal lesion-physiology study of Tomita et al. (1999), which revealed that top-down enhancement signals from the PFC to inferior temporal cortex during visual memory recall are mediated not by subcortical pathways, but front-temporal corticocortical projections and that this modulatory influence is necessary for successful memory recall. This supports the assertion that representations are stored in posterior sensory regions and top-down signals from the PFC trigger the activation of these memory representations (Miyashita 2004). Coupled with the results of lesion-behavior studies (Hasegawa, Fukushima, Ihara et al. 1998) and functional neuroimaging studies (Lee et al. 2002; Ranganath, Johnson and D’Esposito 2003), these results establish a role of PFC-mediated top-down modulation in long-term memory. Recent lesion-physiology studies in rodents have also revealed the presence of modulatory PFC influences on the activity of hippocampal place cells (Kyd and Bilkey 2003) and perirhinal neurons during a spatial delayed-response task (Zironi, Iacovelli, Aicardi et al. 2001).
In humans, combined lesion-ERP studies have provided evidence of PFC-dependent top-down enhancement of visual association cortex activity occurring in the first few hundred milliseconds of the visual processing for selectively attended stimuli (Barcelo, Suwazono and Knight 2000). Moreover, electrophysiological alterations accompanying PFC lesions were associated with deficits in visual detection ability. Comparable findings of PFC-mediated ERP enhancement and performance dependence have been obtained during a selective attention auditory task (Knight, Hillyard, Woods et al. 1981). There is also evidence in humans that the PFC exhibits suppressive control over distant cortical regions. For example, ERP studies in patients with focal PFC damage have revealed that auditory (Knight, Scabini and Woods 1989) and somatosensory (p.206) (Yamaguchi and Knight 1990) evoked responses are enhanced, suggesting disinhibition of sensory flow to these regions. These suppressive influences have also been extended to emotionally salient stimuli, as was recently demonstrated by enhanced ERPs recorded in response to mildly aversive stimuli in patients with orbitofrontal lesions (Rule, Shimamura and Knight 2002). Furthermore, there is evidence that PFC-mediated suppression extends to selectively ignored auditory stimuli (Chao and Knight 1998; Knight et al. 1981).
We are currently evaluating the causal role of the PFC in top-down modulation of activity in the visual association cortex by using the same selective working memory visual task in patients that have had a stroke to different regions of their frontal cortex. Although still preliminary, in comparison to control subjects, patients with damage to the left or right middle frontal gyrus, or to projections connecting the MFG with posterior regions, exhibit impaired top-down modulation of visual processing (Cooney, Gazzaley and D’Esposito 2005). In contrast, a patient with intact MFG exhibited robust top-down modulation despite damage to left insula, inferior frontal gyrus and premotor areas. These data suggest a direct role of the middle frontal gyrus in the visual cortex activity modulation we have recently characterized. Due to the limitations of this lesion technique, in terms of inability to control the anatomical localization of the lesions and the potential for plasticity in response to a chronic lesion, we have now completed a pilot study to evaluate the potential of using transcranial magnetic stimulation (TMS) to study the PFC role in top-down modulation. The goal of this experiment was to utilize repetitive TMS (rTMS) to transiently disrupt neural activity in prefrontal areas identified with fMRI in young adults performing our cognitive task, and then to evaluate cognitive performance and neural electrical measures in distant visual association cortex with EEG during the period of disruption, thus allowing the direct evaluation of prefrontal pathways in top-down modulation and cognition. The pilot experiment performed on four subjects revealed that transient disruption of fMRI identified regions in the middle frontal gyrus resulted in an alteration of distant neural measures of top-down modulation (amplitude increase of the p300 in the remember condition), as well as a significant reduction in speed of the response time on the working memory task. A complete study is now underway to directly evaluate the causal role of the PFC in goal-directed modulation of visual cortex activity (Miller, Gazzaley, McEvoy et al. 2005).
Conclusions
In summary, it is likely that such parallel enhancement/suppression control entails large-scale neural networks (Knight 1997), including an inhibitory PFC-thalamic gating network and a direct excitatory PFC projection to specific cortical regions. Alternatively, suppression might entail long-range excitatory prefrontal-cortical projections that then activate local inhibitory neurons (Carr and Sesack 1998), or perhaps involves the withdrawal of excitatory influences by the reallocation of resources. For a review of computational models of inhibitory control, see Houghton and Tipper (1996). Clearly more empirical research is needed to further our understanding of the mechanisms of top-down enhancement and suppression, as well as place these modulatory control mechanisms within the framework of PFC functional architecture and associated neural networks.
References
Bibliography references:
Awh, E and Jonides, J (2001). Overlapping mechanisms of attention and spatial working memory.Trends Cogn Sci, 5(3), 119–126.
Baddeley, A (1986). Working Memory. Oxford: Oxford University Press.
(p.207) Bar, M (2003). A cortical mechanism for triggering top-down facilitation in visual object recognition. J Cogn Neurosci, 15(4), 600–609.
Barcelo, F, Suwazono, S and Knight, RT (2000). Prefrontal modulation of visual processing in humans. Nat Neurosci, 3(4), 399–403.
Bentin, S, Allison, T, Puce, A, Perez, E and McCarthy, G (1996). Electrophysiological studies of face perception in humans. J Cogn Neurosci, 8(6), 551–565.
Buchel, C and Friston, K (2000). Assessing interactions among neuronal systems using functional neuroimaging. Neural Netw, 13(8–9), 871–882.
Carr, DB and Sesack, SR (1998). Callosal terminals in the rat prefrontal cortex: synaptic targets and association with GABA-immunoreactive structures. Synapse, 29(3), 193–205.
Cavada, C and Goldman-Rakic, PS (1989). Posterior parietal cortex in rhesus monkey: II. Evidence for segregated corticocortical networks linking sensory and limbic areas with the frontal lobe. J Comp Neurol, 287(4), 422–445.
Chafee, MV and Goldman-Rakic, PS (1998). Matching patterns of activity in primate prefrontal area 8a and parietal area 7ip neurons during a spatial working memory task. J Neurophysiol, 79(6), 2919–2940.
Chao, LL and Knight, RT (1998). Contribution of human prefrontal cortex to delay performance. J Cogn Neurosci, 10(2), 167–177.
Chawla, D, Lumer, ED and Friston, KJ (1999). The relationship between synchronization among neuronal populations and their mean activity levels. Neural Comput, 11(6), 1389–1411.
Cooney, JW, Gazzaley, A and D’Esposito, M (2005). Frontal lobe strokes impair top-down modulation of visual processing: fMRI evidence. Society for Neuroscience Abstracts.
Corbetta, M (1998). Frontoparietal cortical networks for directing attention and the eye to visual locations: identical, independent, or overlapping neural systems? Proc Natl Acad Sci USA, 95(3), 831–838.
Corbetta, M, Miezin, FM, Dobmeyer, S, Shulman, GL and Petersen, SE (1990). Attentional modulation of neural processing of shape, color, and velocity in humans. Science, 248(4962), 1556–1559.
Courtney, SM, Ungerleider, LG, Keil, K and Haxby, JV (1997). Transient and sustained activity in a distributed neural system for human working memory. Nature, 386(6625), 608–611.
Craik, FI and Salthouse, TA (2000). Handbook of Aging and Cogntion II. Mahwah, NJ: Erlbaum.
D’Esposito, M, Aguirre, GK, Zarahn, E, Ballard, D, Shin, RK and Lease, J (1998). Functional MRI studies of spatial and nonspatial working memory. Brain Res Cogn Brain Res, 7(1), 1–13.
D’Esposito, M, Deouell, LY and Gazzaley, A (2003). Alterations in the BOLD fMRI signal with ageing and disease: a challenge for neuroimaging. Nat Rev Neurosci, 4(11), 863–872.
D’Esposito, M, Postle, BR and Rypma, B (2000). Prefrontal cortical contributions to working memory: evidence from event-related fMRI studies. Exp Brain Res, 133(1), 3–11.
de Fockert, JW, Rees, G, Frith, CD and Lavie, N (2001). The role of working memory in visual selective attention. Science, 291(5509), 1803–1806.
Desimone, R (1996). Neural mechanisms for visual memory and their role in attention. Proc Natl Acad Sci USA, 93(24), 13494–13499.
Desimone, R and Duncan, J (1995). Neural mechanisms of selective visual attention. Annu Rev Neurosci, 18, 193–222.
Duncan, J, Humphreys, G and Ward, R (1997). Competitive brain activity in visual attention.Curr Opin Neurobiol, 7(2), 255–261.
Eimer, M (2000). Attentional modualtions of event-related brain potentials sensitive to faces.Cognitive Neuropsychology, 17, 103–116.
Friston, K, Phillips, J, Chawla, D and Buchel, C (2000). Nonlinear PCA: characterizing interactions between modes of brain activity. Philos Trans R Soc Lond B Biol Sci, 355(1393), 135–146.
Friston, KJ, Frith, CD, Liddle, PF and Frackowiak, RS (1993). Functional connectivity: the principal-component analysis of large (PET) data sets. J Cereb Blood Flow Metab, 13(1), 5–14.
(p.208) Friston, KJ, Holmes, AP, Worsley, KJ, Poline, JP, Frith, CD and Frackowiak, RSJ(1995). Statistical parametric maps in functional imaging: A general linear approach. Human Brain Mapping, 2, 189–210.
Frith, C (2001). A framework for studying the neural basis of attention. Neuropsychologia, 39(12), 1367–1371.
Funahashi, S, Bruce, CJ and Goldman-Rakic, PS (1989). Mnemonic coding of visual space in the monkey’s dorsolateral prefrontal cortex. J Neurophysiol, 61(2), 331–349.
Fuster, JM (1990). Inferotemporal units in selective visual attention and short-term memory. J Neurophysiol, 64(3), 681–697.
Fuster, JM and Alexander, GE (1971). Neuron activity related to short-term memory. Science, 173(997), 652–654.
Fuster, JM, Bauer, RH and Jervey, JP (1985). Functional interactions between inferotemporal and prefrontal cortex in a cognitive task. Brain Res, 330(2), 299–307.
Gazzaley, A, Cooney, JW, McEvoy, K, Knight, RT and D’Esposito, M (2005a). Top-down enhancement and suppression of the magnitude and speed of neural activity. J Cogn Neurosci, 17(3), 507–517.
Gazzaley, A, Cooney, JW, Rissman, J and D’Esposito, M (2005b). Top-down suppression deficit underlies working memory impairment in normal aging. Nat Neurosci, 8(10), 1298–1300.
Gazzaley, A, Rissman, J and D’Esposito, M (2004). Functional connectivity during working memory maintenance. Cogn Affect Behav Neurosci, 4(4), 580–599.
Hasegawa, I, Fukushima, T, Ihara, T and Miyashita, Y (1998). Callosal window between prefrontal cortices: cognitive interaction to retrieve long-term memory. Science, 281(5378), 814–818.
Hasher, L and Zacks, RT (1988). Working memory, comprehension and aging: A review and a new view. In GH Bower (ed), The Psychology of Learning and Motivation, Vol. 22(pp. 193–225). New York, NY: Academic Press.
Heimer, L (1983). The Human Brain and Spinal Cord: Functional neuroanatomy and dissection guide. New York: Springer Verlag.
Hillyard, SA, Hink, RF, Schwent, VL and Picton, TW (1973). Electrical signs of selective attention in the human brain. Science, 182(4108), 177–179.
Hillyard, SA, Vogel, EK and Luck, SJ (1998). Sensory gain control (amplification) as a mechanism of selective attention: electrophysiological and neuroimaging evidence. Philos Trans R Soc Lond B Biol Sci, 353(1373), 1257–1270.
Holmes, A, Vuilleumier, P and Eimer, M (2003). The processing of emotional facial expression is gated by spatial attention: evidence from event-related brain potentials. Brain Res Cogn Brain Res,16(2), 174–184.
Hopfinger, JB, Buonocore, MH and Mangun, GR (2000). The neural mechanisms of top-down attentional control. Nat Neurosci, 3(3), 284–291.
Houghton, G and Tipper, SP (1996). Inhibitory mechanisms of neural and cognitive control: applications to selective attention and sequential action. Brain and Cognition, 30(1), 20–43.
Ishai, A, Haxby, JV and Ungerleider, LG (2002). Visual imagery of famous faces: effects of memory and attention revealed by fMRI. Neuroimage, 17(4), 1729–1741.
Jha, AP and McCarthy, G (2000). The influence of memory load upon delay-interval activity in a working-memory task: an event-related functional MRI study. J Cogn Neurosci, 12(Suppl 2), 90–105.
Kanwisher, N and Wojciulik, E (2000). Visual attention: insights from brain imaging. Nat Rev Neurosci, 1(2), 91–100.
Kastner, S, De Weerd, P, Desimone, R and Ungerleider, LG (1998). Mechanisms of directed attention in the human extrastriate cortex as revealed by functional MRI. Science, 282(5386), 108–111.
Kastner, S, Pinsk, MA, De Weerd, P, Desimone, R and Ungerleider, LG (1999). Increased activity in human visual cortex during directed attention in the absence of visual stimulation. Neuron, 22(4), 751–761.
Kastner, S and Ungerleider, LG (2001). The neural basis of biased competition in human visual cortex. Neuropsychologia, 39(12), 1263–1276.
(p.209) Knight, RT (1997). Distributed cortical network for visual attention. Journal of Cognitive Neuroscience, 9(1), 75–91.
Knight, RT, Hillyard, SA, Woods, DL and Neville, HJ (1981). The effects of frontal cortex lesions on event-related potentials during auditory selective attention. Electroencephalography and Clinical Neurophysiology, 52(6), 571–582.
Knight, RT, Scabini, D and Woods, DL (1989). Prefrontal cortex gating of auditory transmission in humans. Brain Res, 504(2), 338–342.
Knight, RT, Staines, WR, Swick, D and Chao, LL (1999). Prefrontal cortex regulates inhibition and excitation in distributed neural networks. Acta Psychol (Amst), 101(2–3), 159–178.
Kubota, K and Niki, H (1971). Prefrontal cortical unit activity and delayed alternation performance in monkeys. J Neurophysiol, 34(3), 337–347.
Kyd, RJ and Bilkey, DK (2003). Prefrontal cortex lesions modify the spatial properties of hippocampal place cells. Cereb Cortex, 13(5), 444–451.
LaBar, KS, Gitelman, DR, Parrish, TB and Mesulam, M (1999). Neuroanatomic overlap of working memory and spatial attention networks: a functional MRI comparison within subjects. Neuroimage, 10(6), 695–704.
Lee, AC, Robbins, TW, Smith, S, Calvert, GA, Tracey, I, Matthews, P et al. (2002). Evidence for asymmetric frontal-lobe involvement in episodic memory from functional magnetic resonance imaging and patients with unilateral frontal-lobe excisions. Neuropsychologia, 40(13), 2420–2437.
Lin, FH, McIntosh, AR, Agnew, JA, Eden, GF, Zeffiro, TA and Belliveau, JW (2003). Multivariate analysis of neuronal interactions in the generalized partial least squares framework: simulations and empirical studies. Neuroimage, 20(2), 625–642.
Logothetis, NK, Pauls, J, Augath, M, Trinath, T and Oeltermann, A (2001). Neurophysiological investigation of the basis of the fMRI signal. Nature, 412(6843), 150–157.
Luck, SJ and Hillyard, SA (1995). The role of attention in feature detection and conjunction discrimination: an electrophysiological analysis. Int J Neurosci, 80(1–4), 281–297.
Luck, SJ, Hillyard, SA, Mouloua, M, Woldorff, MG, Clark, VP and Hawkins, H L. (1994). Effects of spatial cuing on luminance detectability: psychophysical and electrophysiological evidence for early selection. J Exp Psychol Hum Percept Perform, 20(4), 887–904.
Luck, SJ and Vogel, EK (1997). The capacity of visual working memory for features and conjunctions. Nature, 390(6657), 279–281.
Makris, N, Kennedy, DN, McInerney S, Sorensen, AG, Wang, R, Caviness, V S, Jr et al. (2004). Segmentation of subcomponents within the superior longitudinal fascicle in humans: A quantitative, in vivo, DT-MRI study. Cereb Cortex.
May, CP, Hasher, L and Kane, MJ (1999). The role of interference in memory span. Mem Cognit, 27(5), 759–767.
McIntosh, AR (1998). Understanding neural interactions in learning and memory using functional neuroimaging. Ann N Y Acad Sci, 855, 556–571.
Miller, BT, Gazzaley, A, McEvoy, K, Knight, RT and D’Esposito, M (2005). Functional deactivation of the prefrontal cortex disrupts posterior physiological signals: Joint TMS/EEG evidence for PFC-mediated top-down modulation. Society for Neuroscience Abstracts.
Miller, EK, Erickson, CA and Desimone, R (1996). Neural mechanisms of visual working memory in prefrontal cortex of the macaque. J Neurosci, 16(16), 5154–5167.
Miller, EK, Li, L and Desimone, R (1991). A neural mechanism for working and recognition memory in inferior temporal cortex. Science, 254(5036), 1377–1379.
Miller, EK, Li, L and Desimone, R (1993). Activity of neurons in anterior inferior temporal cortex during a short-term memory task. J Neurosci, 13(4), 1460–1478.
Miyashita, Y (2004). Cognitive memory: cellular and network machineries and their top-down control. Science, 306(5695), 435–440.
(p.210) Moran, J and Desimone, R (1985). Selective attention gates visual processing in the extrastriate cortex. Science, 229(4715), 782–784.
Niki, H, Sakai, M and Kubota, K (1972). Delayed alternation performance and unit activity of the caudate head and medial orbitofrontal gyrus in the monkey. Brain Res, 38(2), 343–353.
O’Craven, KM, Downing, PE and Kanwisher, N (1999). fMRI evidence for objects as the units of attentional selection. Nature, 401(6753), 584–587.
Penny, WD, Stephan, KE, Mechelli, A and Friston, KJ (2004). Comparing dynamic causal models. Neuroimage, 22(3), 1157–1172.
Pessoa, L, Kastner, S and Ungerleider, LG (2003). Neuroimaging studies of attention: from modulation of sensory processing to top-down control. J Neurosci, 23(10), 3990–3998.
Petrides, M and Pandya, DN (1999). Dorsolateral prefrontal cortex: comparative cytoarchitectonic analysis in the human and the macaque brain and corticocortical connection patterns. Eur J Neurosci,11(3), 1011–1036.
Petrides, M and Pandya, DN (2002). Comparative cytoarchitectonic analysis of the human and the macaque ventrolateral prefrontal cortex and corticocortical connection patterns in the monkey. Eur J Neurosci, 16(2), 291–310.
Pinsk, MA, Doniger, GM and Kastner, S (2004). Push-pull mechanism of selective attention in human extrastriate cortex. J Neurophysiol, 92(1), 622–629.
Ploner, CJ, Ostendorf, F, Brandt, SA, Gaymard, BM, Rivaud-Pechoux, S, Ploner, M et al. (2001). Behavioural relevance modulates access to spatial working memory in humans. Eur J Neurosci, 13(2), 357–363.
Postle, BR, Druzgal, TJ and D’Esposito, M (2003). Seeking the neural substrates of visual working memory storage. Cortex, 39(4–5), 927–946.
Rainer, G, Asaad, WF and Miller, EK (1998). Selective representation of relevant information by neurons in the primate prefrontal cortex. Nature, 393(6685), 577–579.
Ranganath, C and D’Esposito, M (2001). Medial temporal lobe activity associated with active maintenance of novel information. Neuron, 31(5), 865–873.
Ranganath, C, DeGutis, J and D’Esposito, M (2004). Category-specific modulation of inferior temporal activity during working memory encoding and maintenance. Brain Res Cogn Brain Res, 20(1), 37–45.
Ranganath, C, Johnson, MK and D’Esposito, M (2003). Prefrontal activity associated with working memory and episodic long-term memory. Neuropsychologia, 41(3), 378–389.
Rees, G, Frith, CD and Lavie, N (1997). Modulating irrelevant motion perception by varying attentional load in an unrelated task. Science, 278(5343), 1616–1619.
Rissman, J, Gazzaley, A and D’Esposito, M (2004). Measuring functional connectivity during distinct stages of a cognitive task. Neuroimage, 23(2), 752–763.
Rissman, J, Gazzaley, A and D’Esposito, M (2005). The effect of phonological working memory load on top-down enhancement and suppression of visual processing. Society for Neuroscience Abstracts.
Rule, RR, Shimamura, AP and Knight, RT (2002). Orbitofrontal cortex and dynamic filtering of emotional stimuli. Cogn Affect Behav Neurosci, 2(3), 264–270.
Seminowicz, DA, Mikulis, DJ and Davis, KD (2004). Cognitive modulation of pain-related brain responses depends on behavioral strategy. Pain, 112(1–2), 48–58.
Shimamura, AP (1997). The role of the prefrontal cortex in dynamic filtering. Psychobiology, 28(2), 207–218.
Siebert, TM, Gazzaley, A, Rutman, AM and D’Esposito, M (2005). Top-down enhancement of hippocampal-visual association cortex interactions underlies incidental long-term memory. Society for Neuroscience Abstracts.
Silva, L d (1991). Neural mechanisms underlying brain waves: from neural membranes to networks. Electroencephalog Clin Neurophysiol, 79, 81–93.
Skinner, J and Yingling, C (1977). Central gating mechanisms that regulate event-related potentials and behavior. In J Desmedt (ed), Progress in Clinical Neurophysiology, Vol. 1(pp. 30–69). Basel: S Karger.
Sun, FT, Miller, LM and D’Esposito, M (2004). Measuring interregional functional connectivity using coherence and partial coherence analyses of fMRI data. Neuroimage, 21(2), 647–658.
(p.211) Tomita, H, Ohbayashi, M, Nakahara, K, Hasegawa, I and Miyashita, Y (1999). Top-down signal from prefrontal cortex in executive control of memory retrieval. Nature, 401(6754), 699–703.
Treue, S and Martinez Trujillo, JC (1999). Feature-based attention influences motion processing gain in macaque visual cortex. Nature, 399(6736), 575–579.
Ungerleider, LG, Courtney, SM and Haxby, JV (1998). A neural system for human visual working memory. Proc Natl Acad Sci USA, 95(3), 883–890.
Ungerleider, LG, Gaffan, D and Pelak, VS (1989). Projections from inferior temporal cortex to prefrontal cortex via the uncinate fascicle in rhesus monkeys. Exp Brain Res, 76(3), 473–484.
Vuilleumier, P, Armony, JL, Driver, J and Dolan, RJ (2001). Effects of attention and emotion on face processing in the human brain: an event-related fMRI study. Neuron, 30(3), 829–841.
Watanabe, T and Niki, H (1985). Hippocampal unit activity and delayed response in the monkey.Brain Res, 325(1–2), 241–254.
Webster, MJ, Bachevalier, J and Ungerleider, LG (1994). Connections of inferior temporal areas TEO and TE with parietal and frontal cortex in macaque monkeys. Cereb Cortex, 4(5), 470–483.
West, R (1999). Visual distraction, working memory, and aging. Mem Cognit, 27(6), 1064–1072.
Wilson, FA, Scalaidhe, SP and Goldman-Rakic, PS (1993). Dissociation of object and spatial processing domains in primate prefrontal cortex. Science, 260(5116), 1955–1958.
Wojciulik, E, Kanwisher, N and Driver, J (1998). Covert visual attention modulates face-specific activity in the human fusiform gyrus: fMRI study. J Neurophysiol, 79(3), 1574–1578.
Yamaguchi, S and Knight, RT (1990). Gating of somatosensory input by human prefrontal cortex. Brain Res, 521(1–2), 281–288.
Yingling, C and Skinner, J (1977). Gating of thalamic input to cerebral cortex by nucleus reticularis thalami. In J Desmedt (ed), Progress in Clinical Neurophysiology, Vol. 1. Basel: S. Karger.
Zelano, C, Bensafi, M, Porter, J, Mainland, J, Johnson, B, Bremner, E et al. (2005). Attentional modulation in human primary olfactory cortex. Nat Neurosci, 8(1), 114–120.
Zironi, I, Iacovelli, P, Aicardi, G, Liu, P and Bilkey, DK (2001). Prefrontal cortex lesions augment the location-related firing properties of area TE/perirhinal cortex neurons in a working memory task. Cereb Cortex, 11(11), 1093–1100. (p.212)
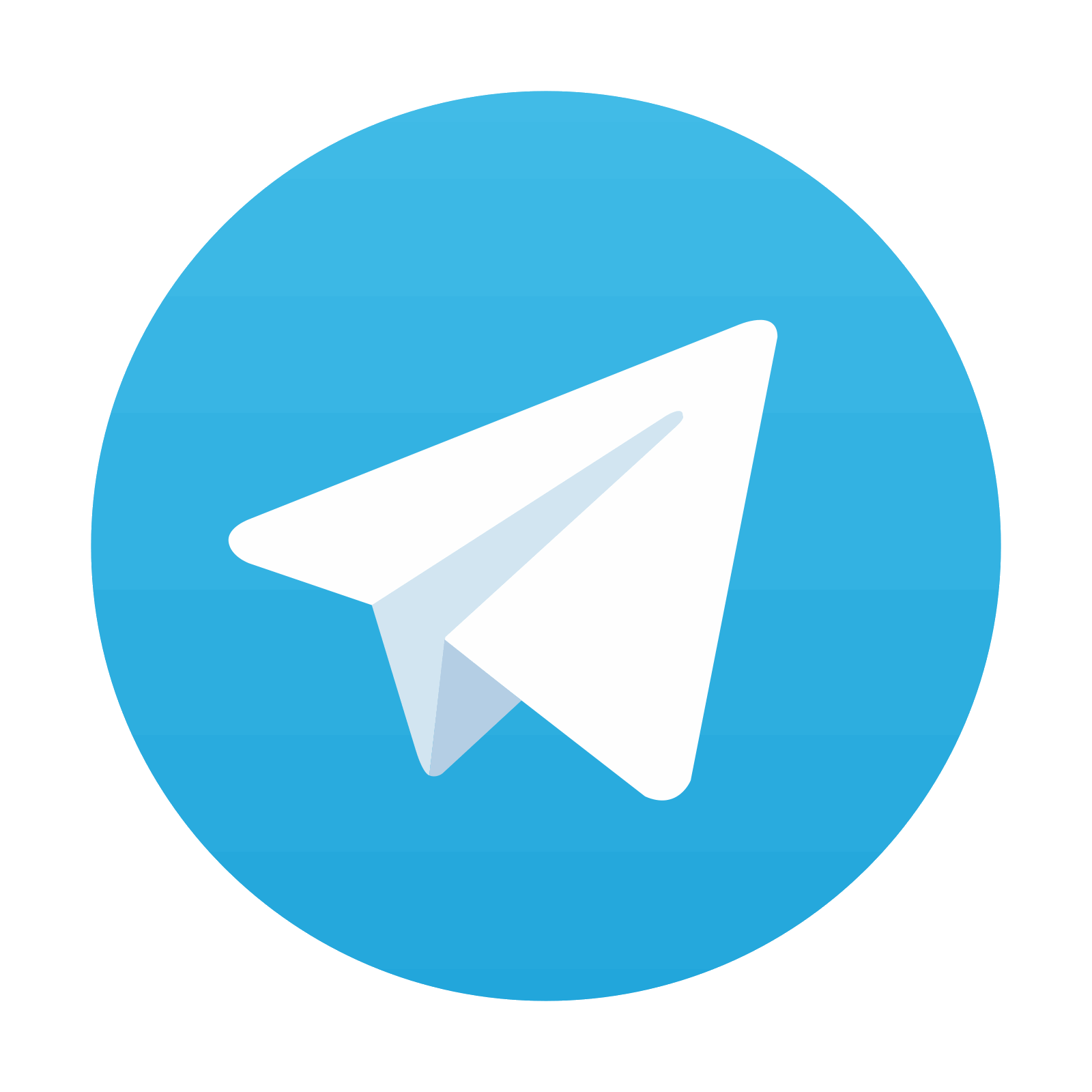
Stay updated, free articles. Join our Telegram channel
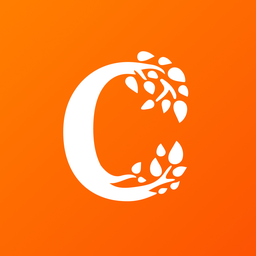
Full access? Get Clinical Tree
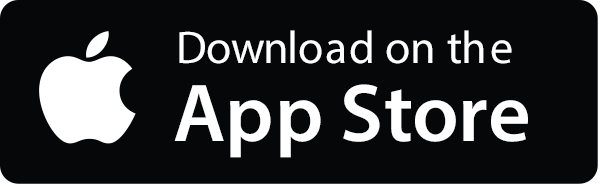
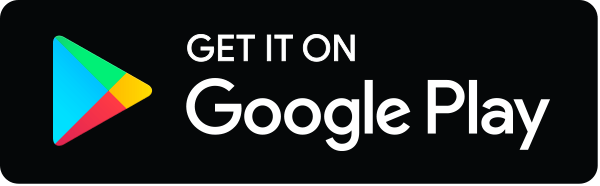