Noninvasive brain stimulation is a valuable investigative tool and has potential therapeutic applications in cognitive neuroscience, neurophysiology, psychiatry, and neurology. Transcranial magnetic stimulation (TMS) is particularly useful to establish and map causal brain-behavior relations in motor and nonmotor cortical areas. Neuronavigated TMS is able to provide precise information related to the individual’s functional anatomy that can be visualized and used during surgical interventions and critically aid in presurgical planning, reducing the need for riskier and more cumbersome intraoperative or invasive mapping procedures. This article reviews methodological aspects, clinical applications, and future directions of TMS-based mapping.
In human brain mapping, 2 basic strategies are commonly used to obtain information about cortical functional representation: (1) recording brain activity during task performance (the passive approach) and (2) observing the effects of eliciting/extinguishing brain activity (the active approach). Techniques using the passive approach include magnetoencephalography (MEG) and electroencephalography (EEG), which provide direct measures of neuronal activity, and positron emission tomography (PET) and functional magnetic resonance imaging (fMRI), which capture brain hemodynamic and metabolic responses as indirect measures of neuronal activation. For the most part, such approaches fail to provide information about causal relationships between certain cortical regions and behavior or cognition. Furthermore, all these techniques are generally based on changes in brain activity that occur during task performance, and therefore depend on collaboration of the individual and careful behavioral assessments. Resting-state fMRI or EEG measures are valuable, novel approaches to studying brain connectivity and network activity, but their usefulness in cortical output mapping is unclear. On the other hand, the active approach minimizes dependency on the individual’s cooperation, because external stimuli are used to elicit or extinguish brain activity, although state-dependent influences on the effects of and responses to brain stimulation need to be considered and controlled for. The active approach investigates whether a specific region of the brain is critical for implementing particular cognitive or behavioral functions and therefore is able to answer questions about causal relationships between brain and function. Noninvasive techniques using such an approach include transcranial electric stimulation (TES) and transcranial magnetic stimulation (TMS).
The noninvasive approach has the advantage of having a greater safety profile and low cost burden for the patients (detailed safety considerations are discussed in later sections). Because of the noninvasiveness of such approaches, they can be repeated as desired, and because stimulation-naive patients get acclimatized to such stimulation techniques quickly, the stress confounders are limited. There is also the added benefit of the lack of medication confounders because most of the noninvasive approaches are based on the underlying activation threshold, thus controlling for the effects of medications on cortical excitability. However, in recent years brain-mapping studies using the passive approach have outnumbered those using the active approach. This situation has been in part because of the problems in correlating the site of stimulation noninvasively to the stimulated cortical region and in part because of the lack of focality of the available noninvasive stimulation devices. The advent of navigated TMS (nTMS) systems has significantly increased the usefulness of TMS in cortical mapping. Such systems can be easily recalibrated after patient movement and, during each stimulation train, the angle and the position of the coil on the scalp can be held constant as verified by real-time visual guidance using the navigation. Using navigation, TMS is able to provide precise information related to the individual’s functional anatomy that can be visualized and used during surgical interventions and critically aid in presurgical planning, reducing the need for riskier and more cumbersome intraoperative or invasive mapping procedures. This article reviews the methodological aspects and clinical applications of noninvasive, brain-stimulation-based mapping.
Historical background
The realization that stimulating/mapping brain areas can guide surgical interventions dates back to Hughlings Jackson, who speculated that the cortex around the central sulcus contained an organized representation of body movements. He suggested that there was a discrete representation of movements of different body parts in this area, and that irritation could produce movements of the corresponding part of the contralateral body. This notion was later confirmed by Fritsch and Hitzig, and then by David Ferrier in the 1870s, who showed that electrical stimulation of the central area in dogs and monkeys could produce movements of the opposite side of the body. Bartholow performed the first stimulation of the human motor cortex a few years later in a patient whose cortex was exposed by a large ulcer on her scalp. This cortical organization was later popularized in the now familiar motor homunculus drawn by Penfield and coworkers ( Fig. 1 ).

The work of Penfield and colleagues established invasive cortical mapping as a standard tool to investigate cortical organization. However, the limited resolution of the early cortical surface stimulation techniques led to the development of better and more advanced stimulation methods such as intracortical microstimulation (ICMS). Nevertheless, all such techniques remained invasive. With the advancement in noninvasive methods of brain stimulation, techniques such as TMS can now be used to leverage the same principle but do so noninvasively, with minimal discomfort to the patients.
Noninvasive brain stimulation provides a valuable tool for interventional neurophysiology applications, modulating brain activity in a specific, distributed, corticosubcortical network so as to induce controlled and controllable manipulations in behavior, as well as for focal neuropharmacology delivery, through the release of neurotransmitters in specific neural networks and the induction of focal gene expression, which may yield a specific behavioral effect. Noninvasive brain stimulation is also a promising treatment of a variety of medical conditions, and the number of applications continues to increase with the large number of ongoing clinical trials in a variety of diseases. Therapeutic usefulness of noninvasive brain stimulation has been claimed in the literature for psychiatric disorders, such as depression, acute mania, bipolar disorders, hallucinations, obsessions, schizophrenia, catatonia, posttraumatic stress disorder, or drug craving; neurologic diseases, such as Parkinson disease (PD), dystonia, tics, stuttering, tinnitus, spasticity, or epilepsy; rehabilitation of aphasia or of hand function after stroke; and pain syndromes, such as those caused by migraine, neuropathies, and low-back pain; or internal visceral diseases, such as chronic pancreatitis or cancer. Although such claims are insufficiently supported by clinical trial data, the potential significance of noninvasive brain stimulation is huge, affecting a large number of patients with debilitating conditions.
In the realm of cortical mapping, the 2 most commonly used techniques for noninvasive brain stimulation are TES and TMS ( Fig. 2 ). Such stimulation of the corticobulbar and corticospinal tracts within the brain are used for intraoperative monitoring and for extraoperative diagnostic assessment of central motor pathways. TMS is more useful for extraoperative diagnostic motor-evoked potential (MEP) studies as well as research applications to establish and map causal brain-behavior relations in nonmotor cortical areas. Both TES and TMS depolarize neurons by producing an electrical current within brain parenchyma, although the ways in which these intraparenchymal currents are generated are different. The advantage of TMS is that it does not activate scalp pain fibers as strongly as TES, and it is therefore useful for assessing central motor pathways in conscious individuals. Furthermore, TMS can also be applied to nonmotor regions in the brain convexity. This article focuses on the methodological aspects and clinical application of TMS in cortical mapping.

TMS
TMS mapping of cortical motor areas follows the basic principles of Penfield, and is based on the idea of stimulating different regions of the brain and measuring the modulatory effect (which can be either excitatory or inhibitory). TMS is a noninvasive technique that uses magnetic stimulation to generate electrical current in the cerebral cortex via a device that generates a brief electric current in a coil placed near the patient’s head. The electric current in the coil in turn creates a magnetic-field variation of 1.5 to 2 T, which penetrates the skull to about 1.5 to 2.0 cm and reaches the brain. The magnetic field then produces currents changing at rates up to 170 A/μs and induces electric fields in the cortex of up to about 150 V/m. Thus, via electromagnetic induction, TMS painlessly induces ions to flow in the brain without exposing the skull to an electric current.
TMS with a focal figure-of-eight coil can be used to show the gross somatotopy of the motor homunculus. Stimuli are applied at various scalp sites using a latitude/longitude-based coordinate system referenced to the vertex, and the amplitude of MEPs, recorded via electromyography (EMG), evoked in contralateral muscles is measured. This process gives a map of sites on the scalp from which responses can be obtained in each muscle of interest. Specifically, the MEP amplitude data recorded at discrete sites over the motor cortex are transformed to a continuously defined function such that the intermediate values are estimated (see article by Thickbroom on mapping elsewhere in this issue for details). The target muscle representation then has a maximum value (optimal site), a center of gravity (CoG) (elements of the representation exceeding 50% of the maximum are used to form a weighted average of their location, in which the weights are given by the normalized value of the element), and a surface area (area where amplitude exceeds 50% of maximum). One of the advantages of this mapping technique is that the optimal site is determined by data from multiple sites, rather than selecting 1 site of largest response. This method has a resolution sufficient to distinguish the optimal site for 2 muscles within the same hand.
Mapping performed with TMS can thus be used to reveal the size of the corticospinal representation of a particular muscle at a given stimulus intensity. As cortical representation increases, the current depolarizes a greater number of cortical cells (in part because of increased current spread), resulting in a steeper curve. Changes in motor cortical maps are also reflected in changes in the slope of stimulus-response curves. Increased excitability of the corticospinal projection is evident from larger MEPs, resulting in a steeper slope of stimulus-response curves and greater area of the representational map.
TMS has developed into a technique that allows the closest noninvasive approximation to electrical cortical stimulation. There have been numerous general reviews of the technique and of the potential for TMS in studies and treatment in neurorehabilitation. Although modeling TMS effects on the brain is an area of active research, the current standard approach is to examine simple maps of responses and determine the CoG and a metric of map size. The CoG is a useful metric because it gives each location stimulated a weight based on the size of the response there. Because the CoG is the result of so many data points, it has a low standard error and high degree of reproducibility. It can be determined with millimeter accuracy, but has no bearing on the spatial extent of the representation. For that purpose, the map volume is often used, which is a sum of the average MEP at each location stimulated, normalized to the average MEP at the location of the largest response. The map volume thus varies from 1, indicating a response at only 1 location, to N, where N is the number of locations in which any response is measured. Stimulation is generally performed at a fixed percentage of the motor threshold, the stimulus strength that elicits measurable MEP in at least half the stimulations. Map volume can be a confusing term, because it refers to the volume of a contour graph constructed on the scalp surface, but represents the area in which stimulation evokes a response.
The area of the map is more difficult to interpret because the site of stimulation with TMS is considerably less focal than that excited via electrodes placed on the cortical surface. The area of a TMS map is therefore a function of both the area of the underlying corticospinal map and the distance from the coil that corticospinal neurons can be activated. One consequence of this situation is that the higher the intensity of the TMS stimulus, the larger the area of the MEP map. In addition, the higher the excitability of the cortical neurons, the easier it is to stimulate them at a distance from the coil. Again, the apparent area of the MEP map is larger than if excitability is low. Levels of excitability are particularly problematic in mapping studies that are performed in individuals who are at rest. The excitability of the corticospinal system in individuals at rest is ill defined: neurons can be quiescent because they are 1 mV from firing threshold or because they are 10 mV from threshold. In the former case, excitability is higher and the MEP map larger than in the latter. In addition to cortical excitability, the area of MEP maps also depends on the excitability of spinal mechanisms. Mapping of the patient typically takes place with the individual at rest, the coil placed tangentially on the scalp with the handle pointing backward and perpendicular to the central sulcus ( Fig. 3 ). After determination of resting motor threshold of the small hand muscles, stimulation is performed at stimulation sites approximately 2 mm apart over an array centered on the central sulcus.

At times, the induced electrical charge after single-pulse TMS is often insufficient to disrupt cortical activity. Instead, repetitive TMS (rTMS) with fast repetition rates is necessary to map these functions. rTMS provides a new window into brain function by creating transient deficits in normal individuals. The higher the stimulation frequency and intensity, the greater is the disruption of cortical function during the train of stimulation. However, after such immediate effects during the TMS train itself, a train of repetitive stimulation can also induce a modulation of cortical excitability. This effect may range from inhibition to facilitation, depending on the stimulation variables (particularly frequency of stimulation). Lower frequencies of rTMS, in the 1-Hz range, can suppress excitability of the motor cortex, whereas 20-Hz stimulation trains seem to lead to a temporary increase in cortical excitability.
Advantages of nTMS
Recent advances in image processing have allowed the refinement of current TMS-mapping strategies by combining MRI modalities with TMS using a three-dimensional (3D) digitizer to measure the position of the stimulating coil and map this position onto an MRI data set. A frameless stereotactic system (FSS) that is rigidly fixated to the stimulating coil is used to correlate scalp stimulation sites to the underlying brain anatomy in real time ( Fig. 4 A). The anatomic accuracy as provided by MRI is combined thereby with the functional motor specificity provided by TMS to introduce stereotactic or nTMS as a new brain-mapping modality. The accuracy of this new technique has been validated by correlating nTMS maps to cortical output maps obtained with direct electrical cortical stimulation (DECS) and to fMRI motor output maps. In addition to the structure-function correlation of nTMS, this technique further allowed for integration of different brain-mapping methodologies by providing a common coordinate system for fMRI and DECS maps.

Coregistration of anatomic MRI data to the sites of stimulation during the TMS session is obtained with the aid of an FSS. This system consists of a jointed mechanical arm, functioning as a stereotactic 3D digitizer, and a computer workstation. Optical encoders in the arm continually measure the angular position of the arm and transmit this information to the computer-graphics workstation, where the spatial position of the tip of the arm is computed in real time and plotted on the MRI data set of the individual’s head. The individual’s head is spatially coregistered to the MRI by means of fiducial markers that are identified on both the MRI data set after imaging is completed and the head during the TMS session. From those 2 sets of coordinates, a matrix transformation is calculated that allows the computer to display the coordinates of the arm tip on the 3D MRI in real time.
Recent studies have highlighted the usefulness of such real-time navigation in identifying cortical motor representation (see Fig. 4 B). Säisänen and colleagues showed that TMS readily identified the primary motor area when it was impossible to elucidate, from visual inspection alone, where the central region was located. The localization was confirmed via DECS during open brain surgery. The same study showed in another patient in whom both fMRI and nTMS were obtained preoperatively to identify the cortical representation of the tongue muscles, fMRI showed a large cortical region after motor activation of the tongue extending to postcentral areas, whereas nTMS exclusively identified precentral cortical regions. It was hypothesized that the postcentral areas identified by fMRI were caused by sensory coactivation during the performance of the task. This assumption was confirmed during open brain surgery, in which MEPs were elicited only from the precentral cortical areas as previously identified by nTMS.
TMS
TMS mapping of cortical motor areas follows the basic principles of Penfield, and is based on the idea of stimulating different regions of the brain and measuring the modulatory effect (which can be either excitatory or inhibitory). TMS is a noninvasive technique that uses magnetic stimulation to generate electrical current in the cerebral cortex via a device that generates a brief electric current in a coil placed near the patient’s head. The electric current in the coil in turn creates a magnetic-field variation of 1.5 to 2 T, which penetrates the skull to about 1.5 to 2.0 cm and reaches the brain. The magnetic field then produces currents changing at rates up to 170 A/μs and induces electric fields in the cortex of up to about 150 V/m. Thus, via electromagnetic induction, TMS painlessly induces ions to flow in the brain without exposing the skull to an electric current.
TMS with a focal figure-of-eight coil can be used to show the gross somatotopy of the motor homunculus. Stimuli are applied at various scalp sites using a latitude/longitude-based coordinate system referenced to the vertex, and the amplitude of MEPs, recorded via electromyography (EMG), evoked in contralateral muscles is measured. This process gives a map of sites on the scalp from which responses can be obtained in each muscle of interest. Specifically, the MEP amplitude data recorded at discrete sites over the motor cortex are transformed to a continuously defined function such that the intermediate values are estimated (see article by Thickbroom on mapping elsewhere in this issue for details). The target muscle representation then has a maximum value (optimal site), a center of gravity (CoG) (elements of the representation exceeding 50% of the maximum are used to form a weighted average of their location, in which the weights are given by the normalized value of the element), and a surface area (area where amplitude exceeds 50% of maximum). One of the advantages of this mapping technique is that the optimal site is determined by data from multiple sites, rather than selecting 1 site of largest response. This method has a resolution sufficient to distinguish the optimal site for 2 muscles within the same hand.
Mapping performed with TMS can thus be used to reveal the size of the corticospinal representation of a particular muscle at a given stimulus intensity. As cortical representation increases, the current depolarizes a greater number of cortical cells (in part because of increased current spread), resulting in a steeper curve. Changes in motor cortical maps are also reflected in changes in the slope of stimulus-response curves. Increased excitability of the corticospinal projection is evident from larger MEPs, resulting in a steeper slope of stimulus-response curves and greater area of the representational map.
TMS has developed into a technique that allows the closest noninvasive approximation to electrical cortical stimulation. There have been numerous general reviews of the technique and of the potential for TMS in studies and treatment in neurorehabilitation. Although modeling TMS effects on the brain is an area of active research, the current standard approach is to examine simple maps of responses and determine the CoG and a metric of map size. The CoG is a useful metric because it gives each location stimulated a weight based on the size of the response there. Because the CoG is the result of so many data points, it has a low standard error and high degree of reproducibility. It can be determined with millimeter accuracy, but has no bearing on the spatial extent of the representation. For that purpose, the map volume is often used, which is a sum of the average MEP at each location stimulated, normalized to the average MEP at the location of the largest response. The map volume thus varies from 1, indicating a response at only 1 location, to N, where N is the number of locations in which any response is measured. Stimulation is generally performed at a fixed percentage of the motor threshold, the stimulus strength that elicits measurable MEP in at least half the stimulations. Map volume can be a confusing term, because it refers to the volume of a contour graph constructed on the scalp surface, but represents the area in which stimulation evokes a response.
The area of the map is more difficult to interpret because the site of stimulation with TMS is considerably less focal than that excited via electrodes placed on the cortical surface. The area of a TMS map is therefore a function of both the area of the underlying corticospinal map and the distance from the coil that corticospinal neurons can be activated. One consequence of this situation is that the higher the intensity of the TMS stimulus, the larger the area of the MEP map. In addition, the higher the excitability of the cortical neurons, the easier it is to stimulate them at a distance from the coil. Again, the apparent area of the MEP map is larger than if excitability is low. Levels of excitability are particularly problematic in mapping studies that are performed in individuals who are at rest. The excitability of the corticospinal system in individuals at rest is ill defined: neurons can be quiescent because they are 1 mV from firing threshold or because they are 10 mV from threshold. In the former case, excitability is higher and the MEP map larger than in the latter. In addition to cortical excitability, the area of MEP maps also depends on the excitability of spinal mechanisms. Mapping of the patient typically takes place with the individual at rest, the coil placed tangentially on the scalp with the handle pointing backward and perpendicular to the central sulcus ( Fig. 3 ). After determination of resting motor threshold of the small hand muscles, stimulation is performed at stimulation sites approximately 2 mm apart over an array centered on the central sulcus.
At times, the induced electrical charge after single-pulse TMS is often insufficient to disrupt cortical activity. Instead, repetitive TMS (rTMS) with fast repetition rates is necessary to map these functions. rTMS provides a new window into brain function by creating transient deficits in normal individuals. The higher the stimulation frequency and intensity, the greater is the disruption of cortical function during the train of stimulation. However, after such immediate effects during the TMS train itself, a train of repetitive stimulation can also induce a modulation of cortical excitability. This effect may range from inhibition to facilitation, depending on the stimulation variables (particularly frequency of stimulation). Lower frequencies of rTMS, in the 1-Hz range, can suppress excitability of the motor cortex, whereas 20-Hz stimulation trains seem to lead to a temporary increase in cortical excitability.
Advantages of nTMS
Recent advances in image processing have allowed the refinement of current TMS-mapping strategies by combining MRI modalities with TMS using a three-dimensional (3D) digitizer to measure the position of the stimulating coil and map this position onto an MRI data set. A frameless stereotactic system (FSS) that is rigidly fixated to the stimulating coil is used to correlate scalp stimulation sites to the underlying brain anatomy in real time ( Fig. 4 A). The anatomic accuracy as provided by MRI is combined thereby with the functional motor specificity provided by TMS to introduce stereotactic or nTMS as a new brain-mapping modality. The accuracy of this new technique has been validated by correlating nTMS maps to cortical output maps obtained with direct electrical cortical stimulation (DECS) and to fMRI motor output maps. In addition to the structure-function correlation of nTMS, this technique further allowed for integration of different brain-mapping methodologies by providing a common coordinate system for fMRI and DECS maps.
Coregistration of anatomic MRI data to the sites of stimulation during the TMS session is obtained with the aid of an FSS. This system consists of a jointed mechanical arm, functioning as a stereotactic 3D digitizer, and a computer workstation. Optical encoders in the arm continually measure the angular position of the arm and transmit this information to the computer-graphics workstation, where the spatial position of the tip of the arm is computed in real time and plotted on the MRI data set of the individual’s head. The individual’s head is spatially coregistered to the MRI by means of fiducial markers that are identified on both the MRI data set after imaging is completed and the head during the TMS session. From those 2 sets of coordinates, a matrix transformation is calculated that allows the computer to display the coordinates of the arm tip on the 3D MRI in real time.
Recent studies have highlighted the usefulness of such real-time navigation in identifying cortical motor representation (see Fig. 4 B). Säisänen and colleagues showed that TMS readily identified the primary motor area when it was impossible to elucidate, from visual inspection alone, where the central region was located. The localization was confirmed via DECS during open brain surgery. The same study showed in another patient in whom both fMRI and nTMS were obtained preoperatively to identify the cortical representation of the tongue muscles, fMRI showed a large cortical region after motor activation of the tongue extending to postcentral areas, whereas nTMS exclusively identified precentral cortical regions. It was hypothesized that the postcentral areas identified by fMRI were caused by sensory coactivation during the performance of the task. This assumption was confirmed during open brain surgery, in which MEPs were elicited only from the precentral cortical areas as previously identified by nTMS.
Integration of TMS with other brain-mapping techniques
Neuroimaging techniques, commonly used to acquire functional cortical representation, such as fMRI, PET, EEG, or MEG, have limitations. fMRI and PET provide indirect measures of brain activity with low temporal resolution. EEG and MEG lack in spatial resolution. None of these methods can provide true insights into causal relations between brain activity and behavior. However, combining such neuroimaging and neurophysiologic methods with TMS offers unique advantages: (1) MRI or PET activation can guide where to stimulate and (2) real-time EEG recording can guide when to stimulate.
For example, Sack and colleagues used neuronavigated TMS to quantify the interindividual variance in the exact location of human middle temporal complex (hMT/V5+) and the respective TMS target position on the skull of the study participants. These investigators showed that targets for TMS application can be reliably selected by individual activation patterns from an fMRI experiment ( Fig. 5 ). Area hMT/V5+ was identified in individual participants using a motion-mapping paradigm. Anatomic and fMRI data were coregistered with stereotaxic data from the participants’ heads, and TMS was applied to the individually defined stimulation sites. TMS at hMT/V5+ but not at a parietal control site led to a significant reduction of correct motion discriminations in an early (−40 to −30 ms) and a late (130–150 ms) time window. In such paradigms, using individual neuronavigation proves to be an important methodological improvement because, first, the exact location of hMT/V5+ can vary considerably between participants and, second, moving phosphenes, which are used to identify hMT/V5+ functionally, can be produced only in a small percentage of participants. Such a methodological approach enables us to reveal and quantify the interindividual variance in the exact location of the target cortex and the respective TMS target position on the skull of the participants.
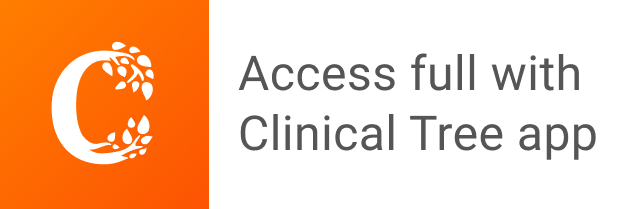