Fig. 12.1
Crystal-Violet-Staining: Histological evaluation at 6 weeks after lateral Fluid-Percussion brain injury. Ventriculomegaly, shrinkage of the hippocampal cell layer, and necrosis is shown
Using another TBI inducing model, the Controlled Cortical Impact (CCI) in mice and rats, histological findings could be confirmed by the detection of substantial tissue loss in the region of impact and selective hippocampal neuronal cell loss have also been observed (Fig. 12.2), and have been related to behavioral deficits seen in this model (Dixon et al. 1999; Riess et al. 2002).
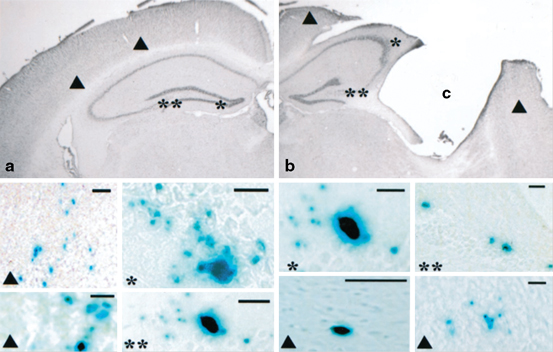
Fig. 12.2
Detection of neural stem cells (NSCs) (C17-2) applying X-gal staining after 13 weeks following transplantation. NSCs were transplanted either contralateral—into the healthy hemisphere- (a) or ipsilateral—into the injured hemisphere- (b). After transplantation into the contralateral hemisphere (a), NSCs were localized in the cortex (▲), dentate gyrus (*) or CA3 (**) region of the hippocampus . After ipsilateral hemispheric transplantation (b), X-gal- cells were located in the cortex (▲) surrounding the injury cavity (C), the granule cell layer of the dentate gyrus (**), and the CA3 region (*) of the hippocampus. (Adapted from Riess et al. 2002)
As the brain has limited capacity for self-repair, mature neurons have no ability to regenerate, and endogenous progenitor cells, although present in multiple locations in the adult mammalian brain, appear to have limited ability to generate new functional neurons in response to injury. For this reason, there is great interest in the possibility of repairing the nervous system by transplanting cells that can replace those lost due to the damage (Bjorklund et al. 2002), or to manipulate endogenous progenitor cells to increase their neurogenic potential or their ability to facilitate regeneration. On the base of current neuropathological knowledge of TBI, a variety of potentially beneficial cellular replacement strategies should be considered. For further information see Schouten et al. (Schouten et al. 2004). One of these options is the use of embryonic stem cells . They show a great potential in the experimental phase on cellular levels as well as in animal trials, but ethical obstacles could be not dismissed in human.
12.3 Embryonic Stem Cells for Transplantation
Embryonic stem cells (ESCs) are nontransformed, pluripotent cells that are derived directly from the inner cell mass of the blastocyst (Evans et al. 1981). These cells are able to participate fully in embryonic development when they were reintroduced into the blastocyst. In vitro, ESCs give rise to cell types of all three primary germ layers in a way that recapitulates events of embryogenesis (Doetschman et al. 1985).
ESCs cultured under specific conditions will differentiate into neuons, astrocytes, or oligodendrocytes, depending on specific factors (Schouten et al. 2004). Due to their ability to differentiate into any tissue-specific cell type, ESCs may therefore possess great therapeutic potential in brain injury. Because TBI is associated with a massive loss of multiple cell types due to primary mechanical tissue disruption, bleeding, and secondary insults such as edema and rise of intracranial pressure leading to cell necrosis. In such case, an entire tissue segment including neurons, glia and vascular structures has to be treated by cells, able to differentiate into all lost cell types (Schouten et al 2004; Riess et al 2007; Molcanyi et al. 2013). Furthermore, ESCs provide a unique cellular system for experimental dissection of lineage specification and determination. Moreover, understanding and controlling ESC differentiation is an important step toward harnessing their potential to differentiate in any cell type of need for biomedical purposes. ESCs have been isolated from mice, monkeys, and humans (Schouten et al. 2004) .
12.4 Host Environment Impairs ESC Survival But Induces ESC Differentiation and Trophic Factor Release
It has been suggested that stem cells hold great potential for the repair of the damaged nervous system. Migration and differentiation of stem cell derived precursors or progenitors seem to be accompanied by an improvement of neurological motor function as well as sensorimotor functional recovery (Riess et al. 2002, 2007). Despite these promising reports, it has to be noted that the number of surviving and differentiating cells after implantation is mostly reduced and therefore cannot completely explain the functional recovery reported. The cell survival and differentiation seems to be not just defined but also restricted by the host environment. This assumption is underlined by reports describing the post-traumatic brain as a hostile environment due to the onset of an acute inflammatory response associated with an activation of immune competent cells, the release of immune mediators, the breakdown of the blood-brain barrier as well as the infiltration of peripheral blood cells and neurotoxic components (Kelly et al. 2004; Lenzlinger et al. 2001). Furthermore, the cellular immune responses, particularly the recruitment of macrophages has been shown to be responsible for loss of cells due to extensive phagocytosis of the transplanted ESCs following TBI (Molcanyi et al. 2007). This potential detrimental effect of the post traumatic brain environment was also shown in vitro. Inflammatory cytokines released following brain trauma such as interferon-gamma (IFN gamma) or tumor necrosis factor-alpha (TNFalpha) seem to inhibit the generation of neurospheres and to exhibit cytotoxic effects on neural stem cells (Wong et al. 2004).
Hence, pathophysiological changes associated with TBI may affect the survival, migration and differentiation of transplanted ESCs (Bentz et al. 2010) examined the effect of trauma associated environmental alterations on stem cell survival and differentiation. They added tissue extract after FP-brain injury (TBE) or healthy rat brains (HBE) to undifferentiated murine embryonic stem cells (CGR8) cultured in feeder-free conditions. Time-dependent survival, proliferation and differentiation of murine ESCs were examined over a period of 7 days. Hereby omission of serum from the culture medium induced neural differentiation of ESCs, as indicated by a significant time dependent down-regulation of oct −4 with a concomitant up-regulation of nestin after 7 days. Pronounced cell loss largely due to apoptotic cell death was observed additionally. In TBE treated cells, on the other hand, a significant amplification of apoptotic cell death, enhancement of nestin and MAP2 expression and marked morphological changes such as axonal-like outgrowth was observed within 3 days of conditioning. Treatment of ESCs with HBE resulted in less pronounced neuronal differentiation processes. Axonal-like outgrowth was not noticed. The pronounced expression of nestin was enhanced in the CGR8 cells following 3 days of incubation with TBE (Fig. 12.3). Furthermore TBE treated cells also expressed Map2. Cells treated with HBE or untreated cells also expressed nestin and Map2. However, analysis of fluorescence intensity revealed significant more intensive nestin and Map2 signals in TBE treated cells than in untreated cells or cells incubated with HBE (Fig. 12.3). The expression of nestin and Map2 confirmed the observed tendency of cells, in particular of TBE treated cells, towards neuronal development.
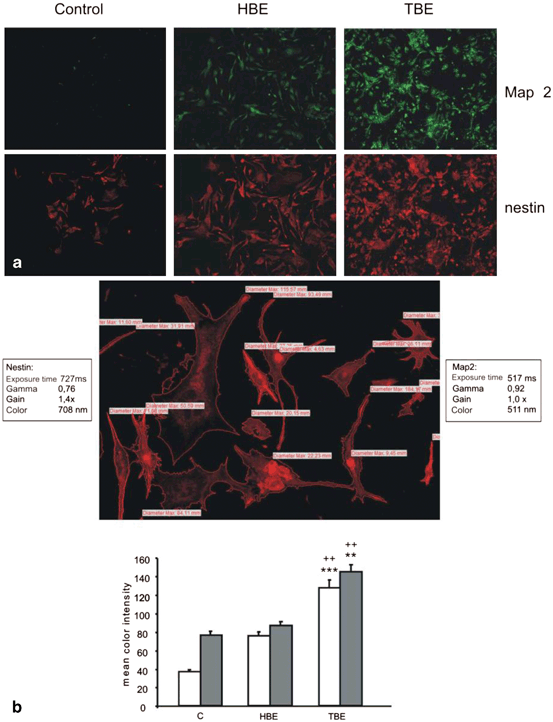
Fig. 12.3
Brain-extract modulation of stem cell differentiation at day 3 following conditioning. CGR8 stem cells were conditioned with brain extract derived from traumatized rats (TBE) or brain extract from healthy animals (HBE). Alternatively, cells were grown in serum-free medium (differentiation control) or under normal/standard conditions (+FCS, +lif). a Expression of nestin (red), and Map2 (green) was assessed by immunocytochemistry. b Quantification of optical density of nestin (white bars) or MAP2 (grey bars) signals were evaluated. Data are presented as mean ± s.e.m of raw data. Significant difference from control (** = p < 0.01; *** = p < 0.001) or HBE conditioned cells (++ = p < 0.01). (Adapted from Bentz K et al. 2010)
In this experiment, the authors suggest that during the early acute phase of traumatic brain injury the cerebral environment is disposed to detrimental as well as potent protective signals that seem to rapidly induce neurogenic processes.
In another experiment (Bentz et al. 2007) compared the capacity of two different ES cell lines to secrete neurotrophins in response to cerebral tissue extract derived from healthy or FP brain injury in rats. The intrinsic capacity of the embryonic cell lines BAC7 (feeder cell-dependent cultivation) to release brain-derived neurotrophic factor (BDNF) or neurotrophin-3 (NT-3) exceeded the release of these factors by CGR8 cells (feeder cell-free growth) by factors of 10 and 4, respectively. Nerve growth factor (NGF) was secreted only by BAC7 cells. Conditioning of cell lines with cerebral tissue extract derived from healthy or brain injured rat brains resulted in a significant time-dependent increase in BDNF release in both cell lines. The increase in BDNF release by BAC7 cells was more pronounced when cells were incubated with brain extract derived from injured brain. Neutrophin-3 and NGF release was inhibited when cell lines were exposed to cerebral tissue extract. The magnitude of the response to cerebral tissue extract was dependent on the intrinsic capacity of the cell lines to release neurotrophins. In this experiment the authors were able to show, that significant variations in the intrinsic capability of different stem cell lines to produce neurotrophic factors exist. In this experiment the authors were able to show significant variations in the intrinsic capability of differ-190 ent stem cell lines to produce neurotrophic factors. Furthermore, a significant modulation of neurotrophic factor release was observed following conditioning of cell lines with tissue extract derived from rat brains.
12.5 ESC Transplantation Following TBI
The cell loss due to TBI based on the primary (mechanical) direct trauma and on the developing oedema leading to apoptosis and necrosis of multiple cell types. Therefore the choice of cell population utilized in replacement therapies after TBI might be critical. Pluripotent ESCs derived from the inner mass of the blastocyst are able to differentiate into any tissue-specific cell type and may therefore possess great therapeutic potential in brain injury, since a variety of cell types are damaged or destroyed following cerebral trauma. In accordance with this assumption pluripotent murine embryonic stem cells (D3 ES cell line) have been shown to survive and differentiate following transplantation into rat brains in an experimental stroke model (Erdö et al. 2003).
Furthermore, enhanced green fluorescent protein (eGFP)-transfected D3 ESCs have been shown to migrate along the corpus callosum to the ventricular walls and to populate the border zone of the damaged brain tissue. They were also found on the hemisphere opposite to the implantation site, indicating the highly migratory behavior of implanted ESCs (Hoehn et al. 2002). In another murine model of TBI (induced by injecting phosphate-buffered saline into the left frontal and right caudal cortex) (Srivastava et al. 2006) analyzed the feasibility of ESC transplantation with the focus on the migration in response to lesions induced in brain tissues, and the mechanism of their in vivo differentiation into neighboring neural cells. They demonstrate that undifferentiated ESCs migrate within 1 week after injection to the damaged regions of brain tissue, engraft, and proliferate. Behavioral assessment was not performed. They conclude that damaged brain tissue provides a niche that attracts ESCs to migrate and proliferate. It has to be taken into consideration that the highly proliferative characteristics (self-renewal) of ESCs combined with the ability to differentiate into all embryonic germinal layers (pluripotency) present a potential threat of tumor development (teratoma, teratocarcinoma) when they are transplanted into the adult CNS. Tumorigenesis has been observed after implantation of undifferentiated human ESCs into healthy rat brains, giving rise to teratomas and malignant teratocarcinomas (Thomson et al. 1998). Accordingly, Erdö et al. (2003) compared the tumorigenic outcome after implantation of D3 ESCs in a homologous (mouse to mouse) vs. xenogeneic (mouse to rat) stroke model. In injured and healthy mouse brains, both transplanted undifferentiated and pre-differentiated murine ESCs produced highly malignant teratomas, while mouse ESCs xenotransplanted into injured rat brain migrated towards the lesion and differentiated into neurons at the border zone of the ischemic infarct. This suggests that tumorigenesis may be related to the host animal rather than to the differentiation status of the implanted cells.
Based upon these findings of Erdö undifferentiated murine ESCs of the D3 line stably transfected with the pCX -(ß-act-) were implanted into the ipsilateral cortex of rats (Fig. 12.5a) 72 h after lateral fluid-percussion brain injury of moderate severity to examine their effects on neurofunctional recovery. The chosen transplantation paradigm based upon reports demonstrating that the initial inflammatory response decreases 3 days after trauma to the brain and the development of astroglial scar begins to build thereafter (Okano 2002). The relatively early time point was chosen in order to avoid the peak of any inflammatory reaction and to allow for the migration and differentiation of stem cells that might be obstructed by the later formation of astroglial scar.
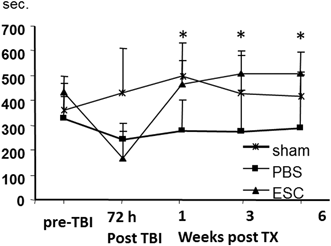
Fig. 12.4
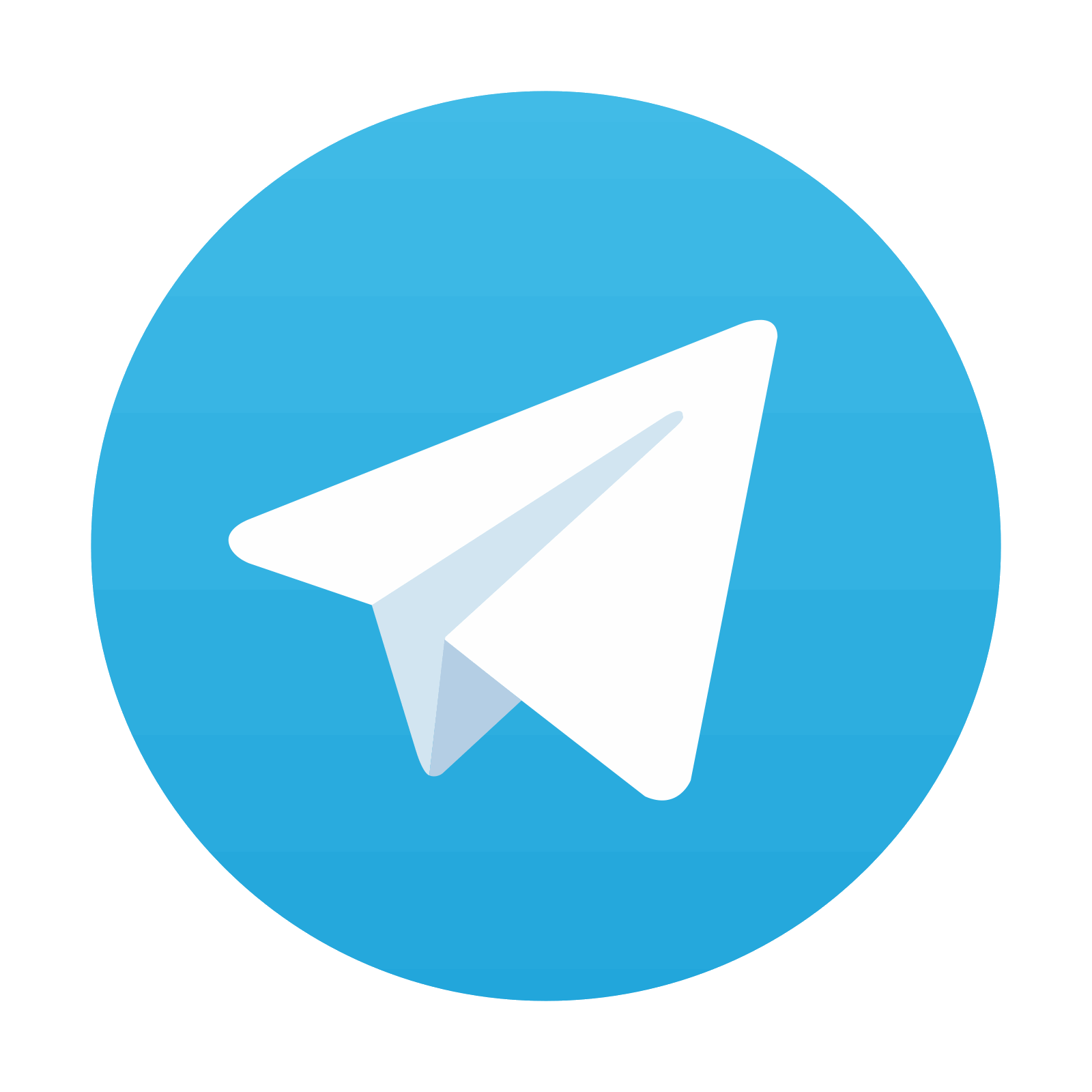
Evaluation of time-dependent modification in locomotive motor function using the rotarod test. *Sham-operated animals. ▲ animals treated with embryonic stem (ES) cells following fluid percussion injury; ■ control animals treated with phosphate-buffered saline (PBS). Times of two performances on the Rotarod were calculated and are expressed as sum ± SE. * p ≤ 0.5 when injured ES-cell transplanted animals are compared to their respective PBS-injected animals. (Adapted from Riess et al. 2007)
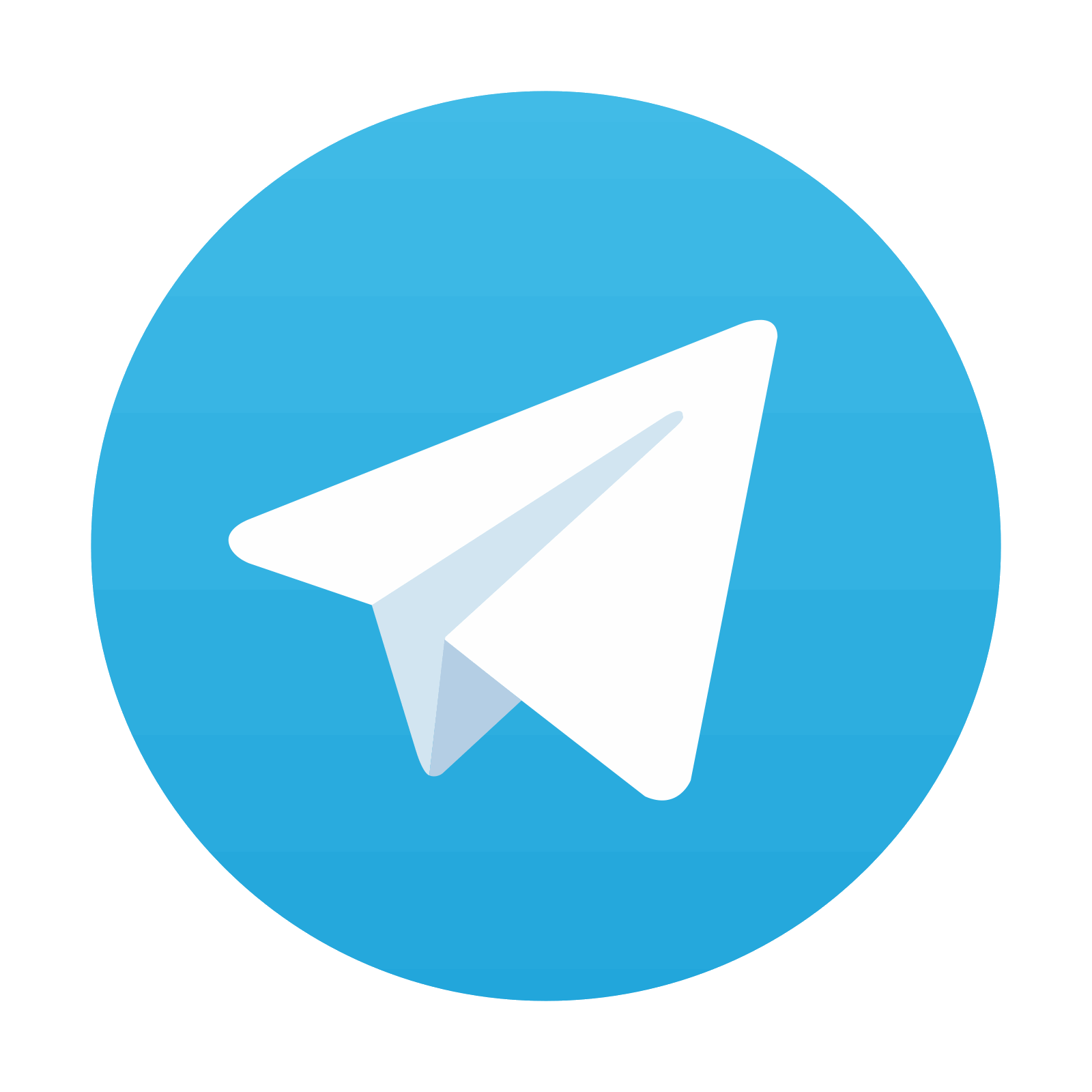
Stay updated, free articles. Join our Telegram channel
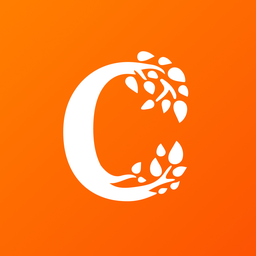
Full access? Get Clinical Tree
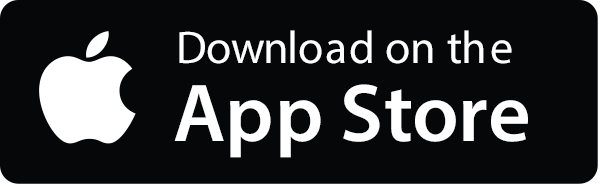
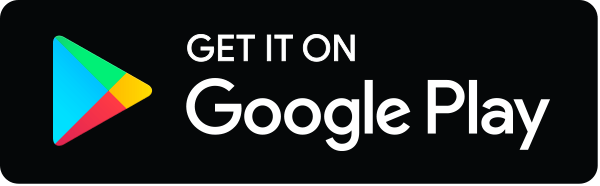
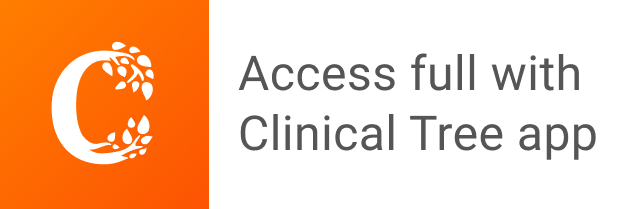