Fig. 11.1
CT examples of findings associated with post-traumatic seizures . (a) Right subdural hematoma. (b) Left frontal contusion. (c) Multiple skull fractures
Other factors that can predispose to the development of post-traumatic seizures include age (incidence is higher in the pediatric population [25] and the elderly [6]), history of alcohol abuse, previous seizures, and a family history of seizures [5]. Genetic predisposition to post-TBI seizures is an interesting issue that has not been well addressed to date. Similar injuries lead to a wide variety of seizure incidence and frequency. Not all studies agree on the genetic predisposition. Jennett found that family history of epilepsy was more common in patients aged less than 16 years with late post-TBI seizures [26]. In the Vietnam Head Injury Study, this factor was not predictive of either early or late seizures [8]. In another study examining genetic susceptibility to epilepsy, seizure incidence among relatives of patients with post-TBI seizures was not higher than among the general population [27]. In a prospective study of late post-traumatic seizures after moderate and severe TBI, 106 patients were examined for the ApoE locus by restriction fragment length polymorphism analysis [28]. Twenty-one patients had at least one late post-TBI seizure. The relative risk of late post-TBI seizures for patients with the ε4 allele was 2.41 (95 % CI 1.15–5.07, p = 0.03). Of note, the presence of this allele was not associated with an unfavorable outcome. Genetic variations of inhibitory neurotransmitters and pathways are also being evaluated as risk factors for post-traumatic seizure development. A 2010 study by Wagner et al. found certain adenosine A1 receptor gene variations to be associated with increased susceptibility of early and late seizures [29]. Darrah et al. found increased risk of post-traumatic seizures between 1 week and 6 months post-injury in patients with a particular polymorphism of the glutamic acid decarboxylase (GAD) 1 gene [30]. Identifying these genetic variations has important implications in both targeting the appropriate patient population to treat with anticonvulsants and helping to determine the pathophysiology of post-traumatic epilepsy .
In studies examining post-traumatic seizures, an arbitrary definition of early and late is commonly used. Early seizures are defined as occurring in the first 7 days after injury and late seizures occur after this point. Early seizure incidence ranges from 2.1 % to 16.9 % [6, 26, 31]. The incidence of late seizures ranges from 1.9 to 30 % [17]. However, this classification is potentially too restrictive. Many TBI patients remain critical in the ICU for longer than 7 days; it would be unreasonable to consider a seizure on the tenth hospital day a “late” seizure. Some studies have extended the acute period to include the first month post-injury. This approach, however, is not ideal, since the underlying cause of seizures in the first week after injury is most likely different from the cause of seizures occurring in the first month or indeed in the first year after injury. Seizures in the first week are more likely related to neurochemical and metabolic derangements, whereas later seizures may be related to the formation of glial scar leading to cortical irritation. Also, there is a significant occurrence of seizures at the scene of both mild and severe TBIs [32, 33]. These immediate seizures are more likely related to the direct disruption of cortical and subcortical connections as a result of percussive forces on the brain and less likely the result of neurochemical or metabolic derangements. However, classifying the timing of onset of post-traumatic seizures is important for both trying to understand their pathophysiology and also in trying to define factors that can predict their occurrence.
A second important goal of dividing seizures by time of occurrence is to evaluate whether early seizures can predict the occurrence of late seizures or the development of a long-term seizure disorder. Early seizures are linked with late seizure development. The increased risk for late seizures after early seizures is independent of the actual number of seizures occurring during the first week after TBI [17]. Not all studies, however, report an increased incidence of late seizures after early post-TBI seizures. A large retrospective study by Annegers et al. utilized multivariate analysis and found that early seizures are not an independent risk factor for late post-traumatic epilepsy , and most likely early seizures are a marker of injury severity sufficient to cause late epilepsy [5]. Another interesting observation is that the incidence of late seizures after early post-TBI seizures is dependent on the age of the patient. Children less than 16 years old may not be at increased risk for late seizures regardless of the early seizure type [5, 17]. Additionally, there is some evidence that immediate seizures occurring at the scene of the trauma are not linked with any increased risk of developing late seizures [32].
The incidence of post-traumatic seizures differs in the pediatric population. The overall incidence is higher than in adults [6, 25, 31]. Early post-traumatic seizures occur slightly more commonly with reported incidence rates of 9–15% [12, 34–36]. As with adults, there is a close correlation between injury severity and the incidence of any type of seizures. Hahn et al. demonstrated that the incidence of post-traumatic seizures was seven times greater in children with severe TBI and GCS <8, compared with milder injury and higher GCS score [34]. Young age is also an important risk factor for seizures, with younger age having higher risk [35–37]. The occurrence of early seizures in severe TBI is also higher than the adult population, with reported incidences as high as 38.7% in one study, which classified severe TBI as GCS 3–8 [35]. Furthermore, early seizures tend to occur even earlier in children; up to 50–80% of early seizures in the pediatric population occur in the first 24 h after injury [25, 38].
Experimental Approaches to Post-traumatic Seizures
Immediate post-traumatic seizures have been described in several experimental models of traumatic brain injury including the impact acceleration model of diffuse TBI, the lateral fluid percussion model [39], and models of cortical contusion [40, 41]. One study monitored EEG for 2 h post-injury in rodents exposed to cortical contusion, and generalized seizure activity was recorded in 14 out of 17 cases, at a mean time of 67 s after trauma [41]. Concurrent microdialysis measured a consistent increase in aspartate, taurine, glutamate, and glycine; however, it is not clear if this represented a cause or a consequence of the seizures. Longer-term behavioral studies in experimental TBI have not demonstrated any significant evidence of clinical seizure activity [42, 43]. This finding is at odds with the clinical behavior of TBI, and it may relate to differences in seizure thresholds between rodents and humans, though the difference may also be explained by the heterogeneity of models of TBI. For example, a study utilizing the lateral fluid percussion model found that nearly half of rats developed clinical epilepsy with a latency of 7 weeks to 1 year post-injury [39]. Another study using the controlled cortical injury and lateral fluid percussion injury models demonstrated that while the rate of delayed seizures is low (3–9%), over 70% of mice had spontaneous epileptiform discharges up to 9 months post-injury with the majority showing increased seizure susceptibility when compared to controls [44]. This ongoing hyperexcitability plays a role in post-traumatic epileptogenesis .
The origin of post-traumatic seizures is not known and improved understanding requires a good experimental model. Studies have evaluated in vivo seizure activity, in vitro seizure activity in brain tissue from injured animals, and in vitro seizure activity in brain tissue injured in vitro. In vivo models have focused on either direct observation of seizures or stimulation of seizures by cortical injection of ferrous chloride [45]; the latter technique is thought to mimic cortical accumulation of blood breakdown products and causes recurrent focal epileptiform discharges. One of the in vitro approaches used isolated hippocampal slices from the brains of injured animals, followed by in vitro electrophysiological recording [46]. In vitro models of traumatic brain injury have difficulty in reproducing a meaningful level of trauma. Models have included scraping hippocampal slices or stretching neurons grown in culture [47, 48]. Combining all these techniques, Golarai et al. examined changes in the rodent brain after weight drop injury to the somatosensory cortex [49]. They found an early selective cell loss in the dentate gyrus and area CA3 of the hippocampus, a persistently enhanced susceptibility to pentylenetetrazole-induced seizures for up to 15 weeks after injury and an abnormal hyperexcitability in the granule cell and molecular layers of the dentate gyrus.
Pathophysiology of Post-traumatic Seizures
The pathophysiological mechanisms causing post-traumatic seizures are not well understood, and there is likely some variation depending on the time of onset after injury. Post-traumatic seizures should be thought of as primary and secondary. Primary post-traumatic seizures are most likely to occur immediately after injury and are caused by direct effects of the brain injury itself. Secondary seizures are caused by other epileptogenic factors not directly related to the injury, such as fever, inflammation, metabolic and electrolyte abnormalities, or drug reactions. Late seizures are explained by structural changes such as neurogenesis and synaptic plasticity [50]. Primary and secondary seizures are related in that the injury itself may contribute to a reduction in seizure threshold, therefore increasing the epileptogenicity of the factors that can cause secondary and late seizures.
A cascade of metabolic and neurochemical events characterize traumatic brain injury, starting at the time of injury and continuing throughout the acute and subacute phases. Many of these changes are potentially epileptogenic, including hyperglycolysis [51]; extracellular elevation of glutamate (and other amino acids, particularly aspartate) [52, 53]; transient flux of ions including sodium, calcium, and potassium [54]; and altered cerebral blood flow [55]. Traumatic brain injury also triggers a series of cellular repair mechanisms such as axonal sprouting, necessary for functional recovery; however, some of these processes may subsequently lead to hyperexcitability and epileptogenesis [56].
In the acute phase, elevations in extracellular excitatory amino acids can cause widespread depolarization that may reach seizure threshold. Loss of inhibitory neurons may promote generation of a seizure focus. Ionic transients may shift the cell membrane equilibrium potential either causing action potential generation or a reduction in the stimulus threshold required to generate an action potential. Changes in extracellular pH can also shift the membrane potential as alkalosis tends to cause depolarization. The most widely studied theory involves the massive rise in levels of the excitatory neurotransmitter, glutamate, which then triggers a series of changes in calcium homeostasis, cell signaling pathways including NMDA receptor activation, and eventually cell death [57].
Seizures arising in the more chronic phases of injury are less likely caused by acute changes in cellular physiology. These late seizures are more likely related to the influence of glial scars, breakdown products of hemoglobin, death of inhibitory interneurons, or disruption of neuronal connections with formation of abnormal neosynapses with greater excitatory potential [58].
A change in the balance of inhibitory and excitatory neurons may also play a role in seizure development. Loss of hippocampal hilar neurons and subsequent hyperexcitability has been identified in injured animals [59]. A phenomenon known as “mossy fiber sprouting ” in which granule cells in the dentate nucleus sprout axons to nearby granule cells forming new connections has been recurrently demonstrated in animal models of post-traumatic epilepsy [39, 60]. These changes occur in the hippocampus ipsilateral to the injury and are correlated with the severity of injury. A pathology study in humans undergoing temporal lobectomy for post-traumatic epilepsy also identified hippocampal cell loss in 94 % of patients and, to some extent, mossy fiber sprouting in all specimens [61]. Using another mouse model of TBI, the neocortical “undercut,” Jin et al. demonstrated that these new connections lead to increased excitatory connectivity upon layer V pyramidal neurons [62]. This cortical reorganization contributes to development of post-traumatic epilepsy.
Epileptogenesis may not be entirely a neuronal phenomenon. It is known that glial membrane channels participate in ionic homeostasis [63] especially at times of neuronal activity. In particular, glial cells buffer levels of extracellular potassium, and failure of this mechanism can result in increased neuronal excitability and seizures [64]. Electrophysiological recordings in hippocampal slices from experimentally injured brains have demonstrated reductions in inward and outward potassium currents and abnormal accumulation of extracellular potassium. Abnormal glial buffering of potassium may represent another mechanism of post-traumatic seizure development [46].
Breakdown of the blood-brain barrier and inflammation are key features of TBI and may also play a role in seizure development. Direct damage to the blood-brain barrier causes increased permeability of foreign substances such as blood proteins, complement, and reactive oxygen species [50]. One study by Seiffert et al. exposed rat cortex to bile salts causing breakdown of the blood-brain barrier and extravasation of serum albumin. Brain slices were then recorded, and abnormal epileptiform activity was seen in 69% of the treated slices compared to only 10% of the sham-operated controls [65]. Focal EEG abnormalities correlated to areas of blood-brain barrier breakdown have also been demonstrated in human brains following injury [66]. Furthermore, a release of cytokines and chemokines occurs within minutes of injury and leads to accumulation of immune cells in the damaged brain and long-term microglial activation [67]. While these inflammatory mediators also play a protective role and are important for neural repair, the complex pathways require further study to determine potential targets for post-traumatic epileptogenesis .
The impact of seizures on macroscopic physiological parameters in traumatic brain injury has not been well studied. In a study using cEEG monitoring in ICU patients with severe TBI [14], the incidence of raised ICP was similar in both seizure and non-seizure patients. The mean ICP was higher in the seizure group (15.6 vs 11.8 mmHg, p < 0.001); however, this finding may simply reflect seizures occurring in the most severely injured patients. A separate analysis comparing ICP values in individual patients on seizure days and non-seizure days demonstrated no significant difference. Furthermore, serial trends of ICP in the hours before and after seizure events did not demonstrate a clear seizure-related effect. Seizures can cause profound hypotension and hypoxia (in the non-ventilated patient), and prolonged myoclonic and tonic-clonic activity can lead to excess tissue and serum lactate levels and acidosis. Moreover, seizures have been found to contribute to altered cerebral metabolism in TBI patients. A study of 34 severe TBI patients found that seizures and periodic epileptiform discharges were associated with lower cerebral microdialysis glucose and elevated lactate/pyruvate ratio indicating metabolic crisis, which then normalized during the non-ictal period [68]. Of particular interest in this study was the finding that periodic discharges, thought by some to be benign, were also associated with metabolic crisis. These factors may constitute secondary insults, known to worsen outcome after TBI [69], and may represent new therapeutic targets.
Diagnosis of Seizures
The occurrence of seizures can be suspected by clinical activity but EEG confirmation is required. The clinical appearance of early seizures in the ICU includes generalized tonic-clonic and focal seizures. Complex partial seizures may also occur, but documentation of these in an intubated, sedated patient is difficult. Focal seizures can appear as rhythmic myoclonic activity or as a more subtle finding, such as a facial twitch [14]. Seizures may also manifest as a decrease in mental status, and any workup for this problem after TBI should include an evaluation for seizures with EEG. Seizures may be masked in the ICU population by the use of neuromuscular blocking agents, and therefore, caution should be taken when using these agents in patients who are at higher risk of post-traumatic seizures and cEEG should be considered.
The EEG can be utilized in different ways for diagnosing post-traumatic seizures. The simplest method is to obtain an EEG in a patient clinically suspected of having seizures. However, this EEG is a snapshot of the injured brain’s electrical activity. If it does not capture a single seizure event or nonconvulsive status, then seizure diagnosis relies on being able to identify abnormal interictal activity. If there is a significant delay in obtaining the EEG, then seizure activity may not be seen and the diagnostic yield of the study is compromised. Few studies have examined the benefit of frequent screening EEGs or cEEG monitoring. Dawson et al. obtained serial short-duration EEGs in 45 brain-injured patients every few days during the first 14 days and found a 25% incidence of seizures by EEG criteria [70]. Vespa et al. examined the role of cEEG monitoring in moderate to severe TBI and found a 22% incidence of seizures among 94 patients admitted to the ICU [14]. More importantly, 52% of the patients with seizures had no clinical evidence of seizure activity. Additionally, in the subgroup with no clinical or electrographic seizures, 10% of patients had epileptiform and nonepileptiform activity. Epileptiform activity included isolated spikes or sharp waves and/or repetitive sharp waves. Pseudoperiodic lateralized epileptiform discharges (PLEDs) were also seen in patients without other evidence of seizures. Nonepileptiform EEG abnormalities observed in these patients included symmetric disorganized slowing, asymmetrical disorganized delta waves with a focus, intermittent rhythmic delta activity, absence of sleep potentials, and progressive loss of EEG amplitude with burst suppression. The latter was associated with impending brain death. Other EEG abnormalities were observed, including increased beta activity, amplitude suppression, and burst suppression. These patterns were seen in both the seizure and non-seizure groups and are related to sedative hypnotics such as midazolam or propofol. Other studies have described a loss of EEG reactivity to external stimuli and loss of spontaneous variability [71–74]. A reactive EEG has been shown to be a good prognostic factor for recovery of consciousness after TBI [75].
Power spectral analysis has also been used to examine prognosis in TBI. Poor prognosis is frequently associated with unvarying activity and a predominance of delta band activity (1–3 Hz) [71]. Additionally, the percent alpha variability (a quantitative EEG trend analyzing the variability in alpha power compared to total power over time) has been evaluated in moderate to severe TBI. Vespa et al. found that patients with reduced percent alpha variability (values less than 0.1) in the first 3 days after injury had a high likelihood of poor outcome, defined as Glasgow Outcome Scale score of 1 and 2, at the time of discharge [76]. Variable spectral patterns are associated with better prognosis [71, 77] as are persistence or return of a peak in the alpha or theta frequency [78, 79]. Figure 11.2 shows examples of various seizures on EEG.






Fig. 11.2
EEG examples of seizures in TBI . (a) Generalized spike and wave activity. (b) Generalized The caption for Fig 11.2 b should be “generalizedseizure” and not “generalized epileptiform discharges. (c) Focal right frontocentral seizure, (i) beginning of seizure with faster frequencies on the right (red), (ii) progression of seizure with increasing amplitude of right-sided discharges, (iii) slowing of right-sided seizure activity, (iv) conclusion of seizure followed by attenuation of EEG
The increased use of cEEG monitoring in intensive care units has led to new recommendations. The Neurocritical Care Society recommends cEEG for all comatose patients, not just limited to intracranial pathology given the high rate of nonconvulsive status epilepticus in critically ill patients. Additionally, cEEG monitoring should be initiated within 1 h of suspected status epilepticus onset, and monitoring should continue for at least 48 h in comatose patients [80]. Similarly, the European Society of Intensive Care Medicine recommends EEG to rule out nonconvulsive status epilepticus in brain-injured patients and for those with unexplained alterations in consciousness who lack primary brain injury [81].
The urgency of ordering EEG when seizures are suspected after TBI has to be considered, as well. In one study, 23 emergent EEGs (EmEEG) , defined as a study performed in less than 1 h from request, were ordered mainly in post-TBI ICU patients [82]. The reason for ordering the test was to rule out convulsive status epilepticus in 12 patients, nonconvulsive status epilepticus in six patients, and seizures in another six patients. Clinical seizures before the test were observed in three patients, and suspicious clinical activity (unclear to the observers if it represented seizures) was observed in 12 additional patients. The EmEEG showed convulsive status epilepticus in three patients , nonconvulsive status in two patients, and epileptiform activity or electrographic seizures in four patients. Half of the patients were already on antiepileptic therapy when the test was performed. As previously mentioned, the emergent need for EEG has been incorporated into the Neurocritical Care Society guidelines for management of status epilepticus [80].
In addition to raw data, the EEG can be analyzed in a variety of ways and is important in identifying and making determinations regarding seizures. Many studies have focused on compressed spectral array and other quantitative methods, whereas the highest yield of information comes from both trend analysis and examination of the raw EEG data. Vespa et al. used frequency analysis by fast Fourier transform in the ICU, followed by examination of two-minute epochs that were evaluated for any increases in total power [14]. Any epoch in which increased spectral power was observed had the raw EEG data analyzed for evidence of seizures. The trend analysis was therefore used to flag periods where seizures might have occurred, and this approach allowed a more focused examination of the raw EEG. While quantitative EEG can be useful and provide additional information, it must be analyzed with caution and only by those skilled in clinical EEG interpretation [83].
Intracranial EEG monitoring is another diagnostic tool to identify seizures that are not visible on scalp EEG. These devices come in the form of subdural strip electrodes or intracortical depth electrodes and have a similar safety profile compared to other brain monitors [84]. Waziri et al. performed intracortical EEG and compared findings to scalp EEG in 14 patients. Ten patients with depth electrode placement had electrographic seizures, and six of these had no corresponding ictal activity on the scalp EEG [85]. A more recent study of 34 severe TBI patients using both surface and depth electrode EEG found a high rate of electrographic epileptiform activity (seizures or periodic discharges) in 21 patients (62%). In nine of these 21 patients, the activity was only visible on the depth electrode [68]. The use of intracranial EEG monitoring may provide a higher sensitivity for detecting post-traumatic seizures.
Treatment of Post-traumatic Seizures
There are two principal goals of treating post-traumatic seizures in the ICU. Firstly, in the acute post-traumatic period, the goal should be rapid cessation of seizure activity in order to prevent secondary physiological and biochemical insults that may lead to worsening injury. Secondly, further episodes of seizure activity should be prevented. There has been some debate about whether prevention of early seizures can result in a lower incidence of late post-traumatic seizures. Studies have also addressed whether long-term prophylaxis with antiepileptic agents is indicated, and this will be discussed below.
The initial management of seizures in the critically ill brain-injured patient should include close observation of vital signs to ensure adequate oxygenation and end-organ perfusion and adherence to ACLS/ATLS guidelines for assessment of ABC (airway, breathing, and circulation). In the non-intubated TBI patient, intubation and mechanical ventilation should be considered depending on the severity of the injury, the duration of mental status change, and the ability to protect the airway [86]. Vasopressors may also be indicated for hemodynamic support. An assessment of the seizure’s origin should also be made. It should not always be assumed that seizures are primarily related to the brain injury. Critically ill patients are exposed to a wide variety of metabolic and pharmacological stressors that may trigger seizures independently of any brain injury. Such stressors include hypoglycemia, hyponatremia, hypocalcemia, hypophosphatemia, hypoxemia, hypocarbia, alcohol/recreational drug withdrawal, fever, infection including meningoencephalitis, and hepatorenal failure. If seizures are newly diagnosed in a brain-injured patient, any of these complicating factors should be evaluated for and corrected as needed. Furthermore, a combination of these factors may interact in an additive fashion with the injury to trigger seizures, where each factor by itself would be insufficient.
Initial pharmacotherapy for seizures is typically in the form of a benzodiazepine . Lorazepam , diazepam , or midazolam are available and should be given as an intravenous bolus. Prolonged seizures may require higher doses or continuous infusions of either midazolam or propofol. Seizures that are recurrent or ongoing despite the above measures should be treated as status epilepticus. If seizures remain refractory , then escalation to barbiturates may be necessary. If an infusion is started, cEEG monitoring should be ordered with a goal of achieving EEG burst suppression (see chapter on Treatment of Status Epilepticus in Critical Care).
A patient not currently on anticonvulsants should be started on one, typically phenytoin or fosphenytoin (15–20 mg/kg IV loading dose and 300–400 mg/day IV or enteral, with frequent assessment of serum levels and a goal of 10–20 mg/dl). Additional agents include levetiracetam (1000–3000 mg IV loading dose and 1000–4000 mg/day IV or enteral), valproic acid (15–20 mg/kg IV loading dose and 600–3000 mg/day IV or enteral), lacosamide (200–400 mg IV loading dose and 200–600 mg/day IV or enteral), or phenobarbital (20 mg/kg IV loading dose and 100–800 mg/day IV or enteral). Phenytoin has been the most widely studied and has benefits over other agents including the ability to load rapidly, the ability to titrate dose to effect, and the widespread ability to follow serum levels. Negative factors against phenytoin are the risk of Stevens-Johnson syndrome , cardiac complications including hypotension and arrhythmias, reliance on hepatic clearance and variability in serum levels due to complex pharmacokinetics, and therefore difficulty targeting the therapeutic range [87]. The newer anticonvulsants such as levetiracetam and lacosamide are now widely available and have minimal drug interactions though these agents are not as well studied in the TBI population.
Several studies have examined the efficacy of anticonvulsants in preventing early seizures. The largest prospective, randomized, double-blind, placebo-controlled trial to date examined 404 brain-injured patients given phenytoin prophylaxis or placebo for 1 year after traumatic brain injury [11]. Follow-up was continued for 2 years and phenytoin levels were maintained in the high therapeutic range. A significant reduction in early seizures (<7 days) was observed. Patients receiving phenytoin had a 3.6% incidence of seizures as compared with 14.2 % in the placebo group (p < 0.001, risk ratio 0.27, 95% CI 0.12–0.62). No significant reduction in the development of late seizures was reported. By the end of the first year, 21.5% of phenytoin-treated patients and 15.7% of the placebo patients had seizures. By the end of the second year, these numbers had increased to 27.5 % and 21.1 %, respectively (p > 0.2). In addition, the incidence of adverse drug effects in the first 2 weeks of treatment was low [88]. Hypersensitivity reactions occurred in 2.5% of the phenytoin-treated patients as compared to 0% of the placebo patients (p = 0.12). Other studies have also confirmed the ability of phenytoin to reduce the incidence of early seizures [89], while others have shown no significant difference [90].
Other anticonvulsants have been evaluated; Glötzner et al. prospectively studied a group of 139 severe TBI patients and found a lower probability of early seizures in the carbamazepine-treated group when compared to placebo (p < 0.05) [91]. There was no difference in late seizures. Temkin et al. conducted another randomized, double-blind study comparing phenytoin to valproic acid for the prevention of post-TBI seizures [92]. In this study, 132 patients were randomized to receive either phenytoin or valproic acid. The early seizure rate was higher on valproic acid; however, the number was small and not statistically significant.
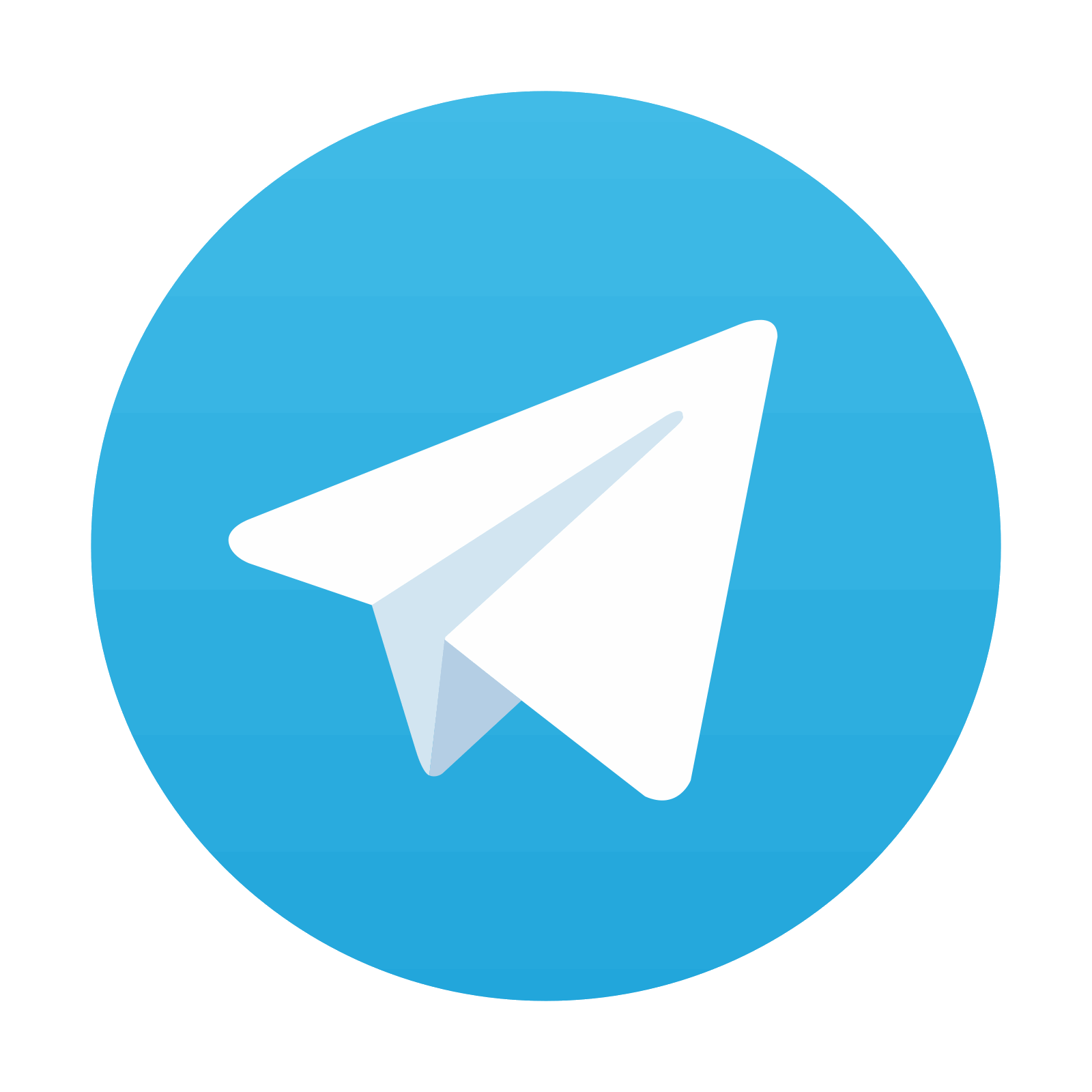
Stay updated, free articles. Join our Telegram channel
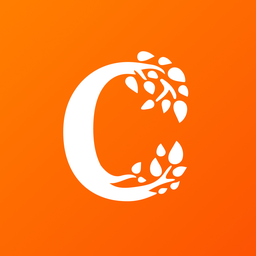
Full access? Get Clinical Tree
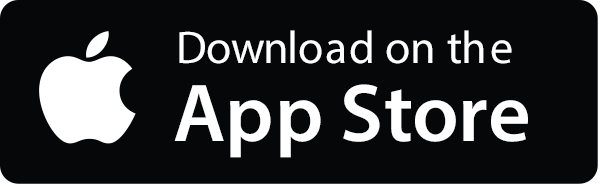
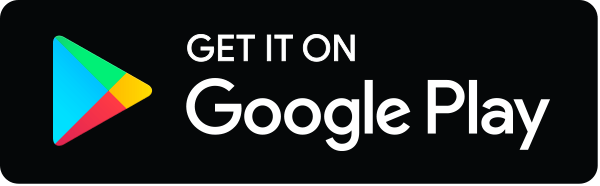