The unique ability to stimulate bilaterally, extracranially, and non-invasively may represent a significant advantage to invasive neuromodulation therapies. In humans thus far the technique has been applied noninvasively, and is termed external trigeminal nerve stimulation (eTNSTM).
Epilepsy affects 3 million Americans, of whom 1 million (30%) have drug-resistant epilepsy. Drug-resistant epilepsy frequently leads to unemployment, injuries, and sudden death. Likewise, major depression is a significant public health problem, affecting more than 17 million American adults, a significant number of whom are persistently ill despite multiple trials of antidepressant medications. Given the side effects of drug therapy for both conditions (eg, sedation, cognitive impairment, behavioral side effects, weight gain and metabolic syndrome, risk for allergies, and suicide), there is a need for alternative nondrug therapies for both epilepsy and depression.
There is growing interest in neuromodulation for depression and epilepsy, including vagus nerve stimulation (VNS), repetitive transcranial magnetic stimulation (rTMS), and deep brain stimulation (DBS) as alternatives to failed drug therapy. These approaches are expensive, have modest efficacy, and can be surgically invasive. Trigeminal nerve stimulation (TNS) is an emerging neuromodulation therapy with unique advantages: it can be delivered externally, bilaterally, and at low cost. Response during a trial of external TNS can be used to evaluate potential surgical candidates before an implantable stimulation device is inserted. This article evaluates the anatomy of the trigeminal nerve, presents animal data, and summarizes recent developments in the application of TNS in drug-resistant epilepsy and major depressive disorder.
Anatomy of the trigeminal nerve
The trigeminal nerve is the largest cranial nerve (fifth or CN V), and has extensive connections with brainstem and other brain structures. The trigeminal nerve has 3 major sensory branches over the face, all of which are bilateral ( Fig. 1 ). These branches pass through 3 foramens in the skull, just lateral to the midline. The nerves within these foramens also supply sensation over the facial structures, and project to the main trigeminal ganglion at the base of the skull. The ophthalmic branch (V1) is ideally placed to allow bilateral stimulation using a single paired electrode placed over the forehead. Fig. 2 details the anatomy of the ophthalmic division of the trigeminal nerve.


The trigeminal ganglion, located in the Meckel cave (cavum trigeminale), projects to the trigeminal nucleus, which has reciprocal projections to the nucleus tractus solitarius (NTS), locus coeruleus, and the reticular formation, structures that play an important role in inhibition of seizures. Evidence from animals indicates that stimulation of the trigeminal nerve and its related structures inhibit seizures. NTS stimulation was investigated in a cat model of epilepsy. NTS stimulation delayed the onset of overt seizures induced by chronic amygdala stimulation (an animal model commonly used to produce chronic epilepsy). The latency to the development of seizures was significantly delayed, and many animals never developed the expected seizures. Overall, intermittent NTS stimulation prolonged or inhibited the onset of kindled seizures. The trigeminal nucleus also has extensive projections to locus coeruleus and midbrain periaqueductal gray, structures involved in the production of the catecholamine neurotransmitters epinephrine and norepineprhine. Stimulation of the locus coeruleus suppresses epileptic discharges induced by cobalt and penicillin, agents used to provoke seizures in animals. The locus coeruleus plays a central role in the anticonvulsant effect of VNS and, given the projections of the trigeminal nerve to the vagus nerve via the reticular formation, the locus coeruleus may share a common role in the efficacy of both VNS and TNS.
Based on animal data linking stimulation of central trigeminal pathways to an antiepileptic effect, Fanselow and colleagues initiated studies of TNS using a rat model of epilepsy, and DeGiorgio and colleagues initiated phase I clinical studies of infraorbital and supraorbital TNS for intractable seizures in humans. In this review, the results of these seminal studies will be presented.
Animal studies
Cranial nerve stimulation has been used for more than a decade to reduce seizure activity in patients with epilepsy. Specifically, the technique of VNS received US Food and Drug Administration approval in 1997 and has been implemented in tens of thousands of patients. However, the mechanisms by which VNS alleviates seizures in responsive patients are not well understood, and not all patients are candidates for, or responsive to, this therapy. Further, it was not previously clear whether the seizure-reduction effect of VNS was specific to the vagus nerve, or whether this effect could be generalized to other cranial nerves.
Therefore, in the laboratory of Dr M.A.L. Nicolelis in the Department of Neurobiology at Duke University, Fanselow and colleagues tested whether stimulation of another cranial nerve, the trigeminal nerve, could exert anticonvulsant effects in awake rats.
Animal studies
Cranial nerve stimulation has been used for more than a decade to reduce seizure activity in patients with epilepsy. Specifically, the technique of VNS received US Food and Drug Administration approval in 1997 and has been implemented in tens of thousands of patients. However, the mechanisms by which VNS alleviates seizures in responsive patients are not well understood, and not all patients are candidates for, or responsive to, this therapy. Further, it was not previously clear whether the seizure-reduction effect of VNS was specific to the vagus nerve, or whether this effect could be generalized to other cranial nerves.
Therefore, in the laboratory of Dr M.A.L. Nicolelis in the Department of Neurobiology at Duke University, Fanselow and colleagues tested whether stimulation of another cranial nerve, the trigeminal nerve, could exert anticonvulsant effects in awake rats.
Methods for stimulating the trigeminal nerve in rats
Initial animal studies of TNS were performed in awake, adult rats using the pentylenetetrazole-induced seizure model. Rats were surgically implanted with arrays of chronic, indwelling electrodes in layer V of the somatosensory cortex and in the somatosensory thalamus. These electrodes allowed for local field potential (LFP) recordings at 16 sites within each recording target. Seizures were initiated using intraperitoneal injection of pentylenetetrazole (40 mg/kg). Injection of this agent induced generalized tonic-clonic seizures with a duration of about 4 seconds and a frequency of 4 per minute for approximately 2 hours.
To reliably activate the trigeminal nerve in awake animals, a nerve cuff electrode, constructed in-house, was implanted around the infraorbital branch of the trigeminal nerve. The nerve cuff electrode contained 2 platinum bands between which current was passed to activate fibers in the infraorbital nerve. This branch of the trigeminal nerve carries somatosensory input from the whiskers on the face of the rat to the trigeminal ganglion, which subsequently projects to the trigeminal brainstem nuclei. These nuclei project to the ventroposterior medial nucleus of the thalamus, which sends afferents to the primary somatosensory cortex.
In these studies, trains of trigeminal stimuli were presented at varying frequencies and amplitudes. Stimulus trains were presented using a continuous duty cycle of 1 minute on to 1 minute off. Seizures observed in the LFP recordings were quantified in 3 ways: (1) number of seizures, (2) seizure duration, and (3) integrated seizure activity, a measure of overall seizure activity calculated by integrating the absolute value of the LFP signals.
Reduction of seizures in animals during TNS
When TNS was applied during pentylenetetrazole administration, seizure activity was reduced in the thalamus and neocortex according to all 3 measures of seizure prevalence. At a stimulus frequency of greater than 100 Hz and a stimulus intensity of 11 mA, a seizure reduction of 78% was observed across all animals. In addition to a reduction in electrographic measures of seizures, as measured in the cortical and thalamic LFPs, the behavioral characteristics of the pentylenetetrazole-induced seizures (clonic jerking of the body and forelimbs) were concomitantly reduced. Thus, the seizure-reduction effect was not specific solely to the somatosensory regions of the thalamus and neocortex.
The seizure-reduction effect was dependent on both stimulus frequency and stimulus intensity. It was necessary to stimulate at greater than 50 Hz to observe a reduction in seizure activity. Increasing stimulus frequency to more than 100 Hz showed no improvement in seizure reduction and, with frequencies less than 50 Hz, there was a trend toward increased seizure duration, peaking at a stimulus frequency of 10 Hz. Seizure reduction increased with increasing current levels, presumably because higher current levels activated more fibers in the stimulated infraorbital branch of the trigeminal nerve. TNS had no discernable effect on heart rate, and animals did not show distress at the levels of stimulation used in these studies.
These initial experiments showed that TNS was effective at reducing pentylenetetrazole-induced seizures in awake animals. We were also interested in 2 other aspects of TNS efficacy against seizures. The first of these was the use of bilateral TNS, and the second was seizure-triggered stimulation.
Efficacy of Bilateral TNS
Unlike VNS, in which typically only the left vagus nerve is stimulated to avoid cardiac side effects, TNS can readily be applied to both trigeminal nerves simultaneously. However, it was not known whether this would improve the efficacy of TNS or whether unilateral stimulation of the trigeminal nerve was sufficient to elicit the full seizure-reduction effect of TNS. Therefore, nerve cuff electrodes were implanted bilaterally on each of the infraorbital nerves along with indwelling electrodes in each of the primary somatosensory cortices. Each infraorbital nerve was stimulated individually and then both nerves were stimulated simultaneously. When both infraorbital nerves were stimulated simultaneously, there was a decrease in the current required to achieve maximal seizure reduction. That is, with unilateral TNS, a stimulus intensity of 11 mA was required to achieve 75% seizure reduction, whereas with bilateral stimulation the same degree of seizure reduction was accomplished with 7 mA of current presented to each nerve simultaneously. This result suggests that the effects of TNS increase with multiple nerve stimulation sites, which has implications for the optimization of TNS application in patient populations. The electrographic (and behavioral) seizure-reduction effects were identical for each side stimulated (ie, either contralateral or ipsilateral to the neocortical recording site). This is further evidence that the effects of TNS involve more than just the brain regions directly targeted by the trigeminal nerve.
Closed-loop Seizure-triggered TNS
An open question about cranial nerve stimulation was whether it was better to provide stimulation on a continuous on-off duty cycle or to apply responsive stimulation only when a seizure was detected. Such a closed-loop paradigm required the development of a seizure-detection algorithm capable of detecting a seizure and promptly triggering TNS. In conjunction with Ashlan P. Reid in the Department of Biomedical Engineering at Duke University, a seizure detector was developed that triggered TNS when the LFP signal increased to more than a manually set voltage threshold. When such activity was detected in the LFP, 500 millisecond trains of 500-microsecond pulses were delivered until the aberrant LFP activity was no longer detected. When unilateral TNS was provided in this seizure-triggered manner, it successfully stopped seizure activity, in an average of 529 milliseconds after seizure onset. This seizure-reduction effect was not observed when TNS was initiated manually after a seizure had been underway for more than 10 seconds (Fanselow, Reid, and Nicolelis, unpublished observations, 2010), so it therefore seemed to be critical to provide the stimulation early in a seizure episode. These results showed that TNS could successfully be applied in a closed-loop, seizure-triggered manner. This finding has implications for the mechanism(s) by which TNS reduces seizure activity. It is likely that, in humans, a more sophisticated seizure-detection algorithm would be required for implementation of a closed-loop stimulation paradigm, but these results serve as a proof of principle for seizure-triggered TNS.
Potential Mechanisms of TNS Seizure-reduction Effects
The mechanisms by which any type of cranial nerve stimulation reduces seizure activity are poorly understood. Multiple studies have suggested that a component of the effect of VNS on seizures is caused by involvement of the neuromodulators released from brainstem nuclei or their downstream targets. For example, Krahl and colleagues showed that lesions of the locus coeruleus, the noradrenergic nucleus of the brainstem, blocked the seizure-suppression effects of VNS. Recent evidence for the involvement of norepinephrine was obtained by Raedt and colleagues who showed that norepinephrine levels in the hippocampus increased during VNS. Whether noradrenergic effects are also present during TNS is not yet known. In addition, the reticular activating system, including the reticular formation of the brainstem, has been implicated in reducing seizure activity, primarily because stimulation of this region is known to desynchronize firing in the neocortex.
It is likely that there are multiple mechanisms by which cranial nerve stimulation, including TNS, exerts its effects on the brain. Evidence for this comes from the different time scales on which cranial nerve stimulation can be effective. First, as discussed earlier, seizure-triggered TNS was able to abort seizures on a rapid time scale, suggesting that TNS can have immediate effects on seizure activity. In support of this, there is evidence that neuronal firing is suppressed during TNS in rats as soon as the nerve stimulation begins. Second, in humans, VNS and TNS are typically provided on a continuous fixed-duty cycle, and, in animal studies, it has been shown that there is a period of increased seizure threshold after termination of each On cycle. Therefore, there may also be effects of cranial nerve stimulation that last from tens of seconds to minutes and outlast the period of nerve stimulation. In addition, there seem to be long-term effects of cranial nerve stimulation, in which seizure frequency and severity are observed to decrease in the course of months to years. This finding suggests that TNS could potentially cause long-term alterations in the brain that could depend on genetically mediated changes in brain function, as has been shown for VNS. None of these seizure-reduction mechanisms are mutually exclusive, and they could operate concurrently to reduce seizure activity.
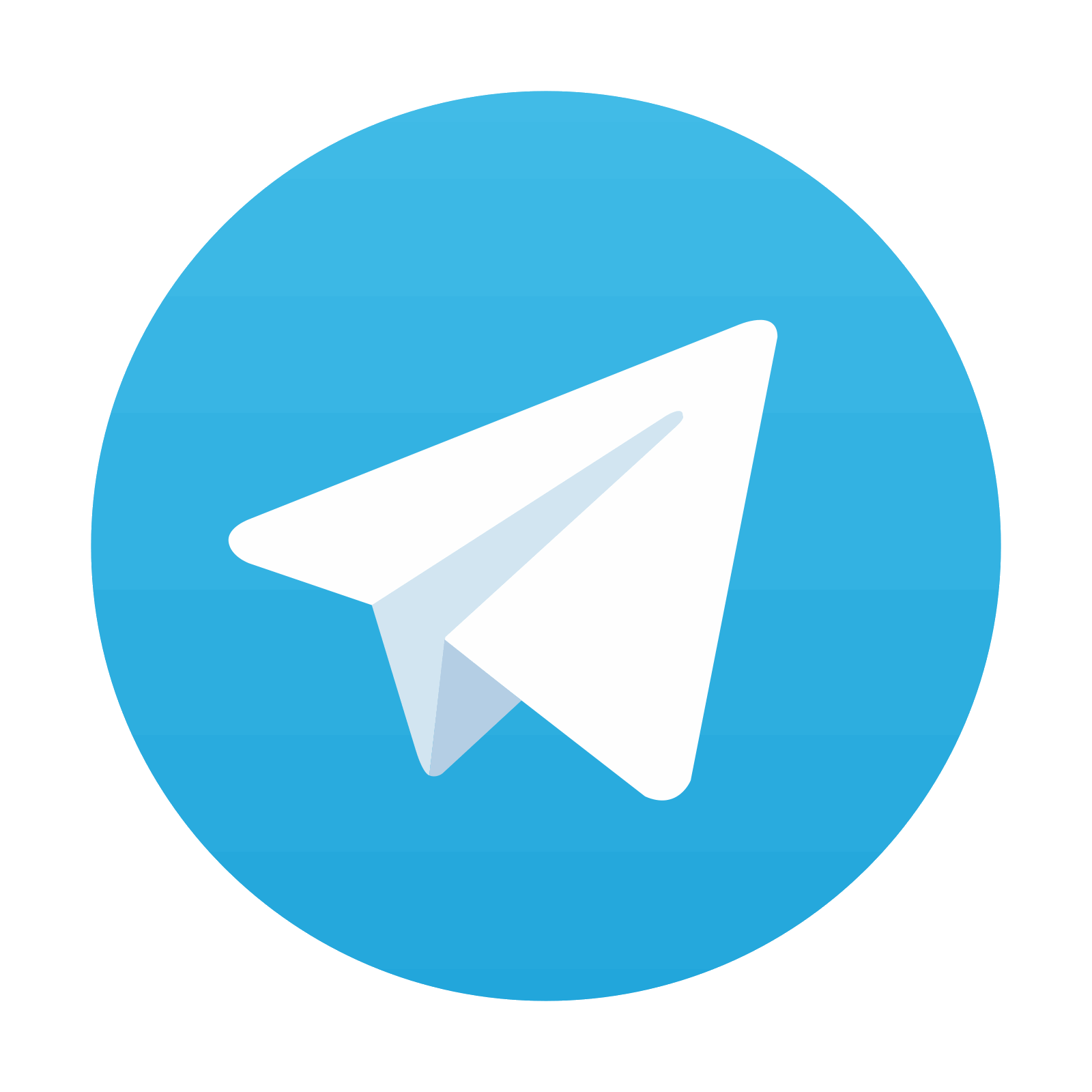
Stay updated, free articles. Join our Telegram channel
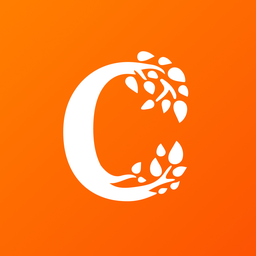
Full access? Get Clinical Tree
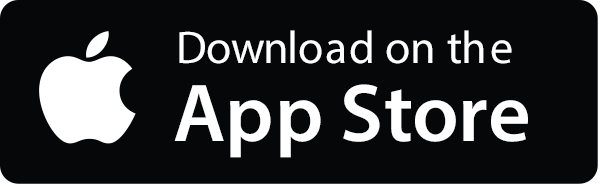
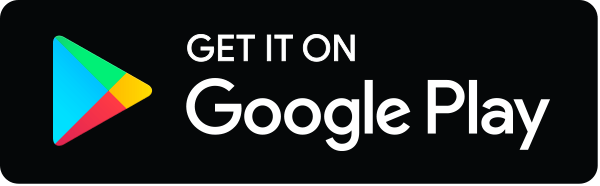