Acknowledgments
The authors acknowledge and thank Kisa Zhang for her artwork in Fig. 17.1 .
Historical context of electric field treatment
The application of physical energy from various parts of the electromagnetic spectrum is common in glioblastoma treatment. The most widely used involves energies from the higher end of the spectrum in exahertz (the 10 18 -Hz range), in which ionizing radiation is used to treat various types of malignancies, including glioblastoma ( Fig. 17.1 ). Therapeutic radiation can be diffuse, as in whole-brain and involved-field radiotherapy, or highly conformal, as in stereotactic radiosurgery (SRS), and the biological responses to these different types of radiation are different, as modeled by the linear-quadratic dose-effect relationship. At the lower end of the spectrum, in the gigahertz or 10 9 -Hz microwave range, laser interstitial thermal therapy (LITT) is being used for the thermocoagulation of brain tumors and the treatment of radiation necrosis. MRI technology now allows the real-time visualization of temperature changes during LITT treatment of a target lesion. In addition, at an even lower part of the electromagnetic spectrum, in the kilohertz or 10 3 -Hz range, alternating electric field therapy or tumor-treating electric fields (TTFields) are now an established treatment for glioblastoma. This chapter provides a summary of the cell biology and physical science effects of TTFields on tumor cells and tumors in the brain, as well as a historical perspective of the clinical studies conducted in the glioblastoma population.

Biological basis and physical science supporting the use of tumor-treating electric fields
Cell Biology Effects of Tumor-treating Fields
Early in vitro studies showed that cells exposed to TTFields underwent violent membrane blebbing during mitosis, thought to be a result of the disruption of alpha/beta tubulin assembly in mitotic spindles. More detailed analysis revealed that these cells seem to transit normally through metaphase, showing normal rates of cyclin B destruction and metaphase exit at times consistent with the expected entry into anaphase. However, anaphase and cytokinesis were perturbed, leading to aberrant mitotic exit. Mitosis is dominated by a myriad of processes that must be regulated spatially and temporally in order to ensure even distribution of parental genomic DNA into the resulting daughter cells. Most of the regulatory events during mitosis control functions that are involved in the migration and alignment of chromosomes, as well as the timing of the contraction of the cytokinetic furrow following chromosome separation. Given the timing of the TTField-induced cellular disruption during mitosis, they likely exert their effect on intracellular proteins that are necessary during late metaphase or anaphase and that bear high electric charges on which the TTFields can act. It is not clear whether these TTField effects are caused by their combined activities on multiple proteins or whether they arise from the perturbation of a single protein that serves a critical function. In order to serve as a TTField target, proteins must possess a sufficiently high dipole moment in order for the alternating electric fields generated by the TTField therapy device to perturb its function in mitosis.
By examining the published dipole moment database of different proteins, the authors found that the heterotrimeric protein complex composed of septin 2, 6, and 7 possesses a dipole moment of 2711 Debyes (or 10 -18 statC·cm SI equivalent), which is 5 standard deviations greater than the median value derived from an analysis of more than 14,000 intracellular proteins. Importantly, this septin complex performs important functions during both metaphase and anaphase and its disruption by short hairpin RNA-mediated depletion resulted in blebbing during mitosis similar to that seen in TTField-treated cells. TTFields are able to perturb normal septin localization during mitosis and cell spreading, which strongly suggests that TTFields physically interact with this complex and exert their effects on mitotic cells by preventing the proper localization of this septin-containing complex to proper sites of action during mitosis ( Fig. 17.2 ). The TTField-induced catastrophe during anaphase results in a failure to complete division that results in a G 0 /G 1 arrest and p53-dependent apoptosis. Therefore, TTFields are likely to exert an effect on multiple proteins and the resulting cumulative perturbation may be necessary to drive the observed mitotic catastrophe.

Postmitotic cells that are treated with TTFields and aberrantly exit mitosis develop aneuploidy, and these aneuploid cells are in general resistant to apoptosis, a process that is known to trigger an immunosuppressive response in the host. However, TTFields also cause cytoplasmic stress and additional signs of immunogenic cell death, including high mobility group box 1 (HMGB1) secretion into the extracellular space, calreticulin upregulation on the cell surface, and annexin V binding. These findings suggest that TTFields may increase the immunogenicity of tumor cells in vivo . When highly metastatic VX-2 tumors were injected into the kidney capsules of rabbits and were treated with TTFields for 7 days after their establishment, metastases to the lungs were markedly reduced compared with nontreated animals. Recovered lung metastases also revealed a significant increase in infiltration of immune cells within the tumors. An interpretation of these results is that TTFields acted to sensitize the animals against metastatic spread and that the increased leukocytic infiltrates reflected an increased requirement for the immunosuppressive stroma for their establishment and maintenance. As discussed later, patients treated with the immunosuppressive steroidal antiinflammatory drug dexamethasone seem not to respond to TTField treatments and those with higher levels of CD3 + , CD4 + , and CD8 + lymphocytes are more likely to have a better outcome on this therapy. Collectively, these data strongly support an immunologic basis for the antitumor response from TTField treatment.
Physical Properties of Tumor-treating Fields and Electric Field Distribution Within the Brain
The physical effects of TTFields are governed by the fundamental physics of Gauss’ law, Ohm’s law, the continuity equation, and Coulomb’s law. In addition, several factors, including a medium’s electrical conductivity (a measure of the ability to pass charges) and relative permittivity (a measure of the ability to hold charges), can affect the electric field distribution within the brain tissue. Because each tissue type is unique, the intracranial structures must therefore be characterized based on their conductivity and permittivity values. The highly heterogeneous consistency and geometry of the brain therefore distort the intracranial electric fields as induced by an external source. Electric fields are generally defined by instantaneous changes in electric potential. These changes in electric potential result in electromotive disruption of mitotic structures and are therefore the basis for the therapeutic benefit of TTFields. TTField therapy for glioblastoma is delivered by 2 pairs of transducer arrays positioned orthogonally on the shaved scalp, adhered by a thin layer of conductive gel that provides good conductivity ( Fig. 17.3 ). TTFields are generated by a battery-powered alternating current generator, operating at 200 kHz with maximum voltage alternating from +50 to −50 V.

To obtain a comprehensive model of the electric field’s distribution in the brain, computer modeling can be performed using coregistered patient DICOM (Digital Imaging and Communications in Medicine) data sets from T1-weighed postgadolinium, T2, and MPRAGE (Magnetization-Prepared Rapid Acquisition with Gradient Echo) MRI. Previously, Lok and colleagues showed a heterogeneous distribution of electric fields in the brain, and the regions adjacent to the ventricular horns had a particularly high electric field intensity ( Fig. 17.4 ). This high field intensity is likely caused by the higher electric conductivity of cerebrospinal fluid than the surrounding tissues, which behaves like the terminal of a capacitor, with the surrounding tissues functioning like a dielectric between conductive terminals. Since a dielectric medium generally retains charge, the rate at which the medium is able to collect and retain the charge is defined by its conductivity and relative permittivity. At 200 kHz, the effect of permittivity is overwhelmed by the conductivity of the medium ( Fig. 17.5 ). Furthermore, each medium has a unique capacitive reactance characteristic of the medium’s conductivity, and the rate at which the medium is able to collect and retain charges is frequency dependent. At high frequencies, the medium only has limited time to collect a finite amount of charges and retain them before the field collapses as the polarity changes direction, thereby discharging the initially retained charges before repeating the process.


Because cerebrospinal fluid has a low permittivity value compared with its surrounding tissues, it is a poor dielectric medium and thus charges migrate through the fluid layer at a much faster rate with minimal charge retention. This property explains why most of the cerebrospinal fluid has very low electric field intensity. But this is not true at the interface between cerebrospinal fluid and its adjacent brain tissue. For a perfect solid conductor, the electric field within it is zero, and the charges are uniformly distributed on the surface of the conductor. However, cerebrospinal fluid is neither a perfect conductor nor a dielectric. When charges are positioned across a parallel flat surface, repulsive forces are generated and the charges dissipate away from each other. However, on surfaces with very steep geometric gradients (ie, sharp corners), the repulsive forces are greatly increased because the charge density is much higher within a smaller surface area. Therefore, it is expected that the ventricular horns have a significantly higher electric field intensity than the rest of the cerebrospinal fluid space as a result of the bunching effect of charge distribution in a region with an irregularly sharp geometry.
The electric properties of gliomas can also vary among patients depending on their tumor composition. Tumors with larger necrotic cores are likely to have higher field intensities in the gross tumor volume because of the capacitive reactance, as explained earlier. In contrast, tumors with smaller or no necrotic core are likely to have lower field intensities at the center of the volume because of absence of an adjacent conductive medium to act as an electric current source. This property may become clinically relevant due to the increased requirement for time of exposure to TTFields as the outer layers of the gross tumor volume are treated by lower field intensities.
Evidence for the use of tumor-treating electric fields in the treatment of recurrent and de novo glioblastomas
First-in-Human Tumor-Treating Fields Therapy for Glioblastoma
The first-in-human pilot trial for safety and efficacy of TTFields therapy was conducted in Europe from 2004 to 2005 and enrolled 10 patients with recurrent glioblastoma. The most common adverse event was contact dermatitis, which occurred in 9 patients and was thought to be a result of hydrogel-induced irritation on the scalp. Two patients experienced partial seizures that were related to their tumors. No toxicity on blood count or chemistry was seen, except for increased liver enzyme levels in those taking anticonvulsants. The median overall survival (mOS) of the 10 patients was 14.4 months. The time to tumor progression was 6.0 months and the 1-year overall survival (OS) rate was 67.5%, which compared favorably with the historical data of 5.8 months for mOS, 2.1 months for median progression-free survival (mPFS), and 21% for 1-year OS. There was 1 complete and 1 partial responder who were alive at 84 and 87 months, respectively, from treatment initiation. Importantly, the intensity of electric fields as directly measured in 1 patient was validated to be within 10% of the values estimated by computer modeling of the electric field distribution within the brain.
A concurrent pilot study was conducted from 2005 to 2007 that enrolled 10 patients with newly diagnosed glioblastoma. TTFields were added to adjuvant temozolomide after initial standard-of-care radiotherapy and concomitant daily temozolomide. The mPFS was 35.8 months and mOS was greater than 39 months, which compared favorably with the mPFS of 6.9 months and mOS of 14.6 months from the data in the phase III trial. The only adverse event noted in the pilot cohort was scalp dermatitis, and that could be ameliorated by topical corticosteroids and periodic shifting of transducer arrays. There were 2 long-term survivors who lived 84 and 64 months from their initial diagnoses.
Tumor-Treating Fields for Recurrent Glioblastoma
The phase III pivotal trial of TTFields for recurrent glioblastoma was conducted from 2006 to 2009 and the primary end point was OS ( NCT00379470 ). In the intent-to-treat population, the median OS was 6.6 months for subjects treated with TTFields versus 6.0 months for those who received best physician’s choice (BPC) chemotherapy, with a hazard ratio of 0.86 ( P = .27) ( Fig. 17.6 ). About 31% of the BPC chemotherapy cohort received bevacizumab alone or in combination with chemotherapy. The mPFS was 2.2 and 2.1 months respectively for TTFields and BPC chemotherapy treatment, with a hazard ratio of 0.81 ( P = .16). The progression-free survival (PFS) at 6 months was 21.4% and 15.1%, respectively ( P = .13). The 1-year survival rate was 20% in both cohorts. The outcome of the trial indicated that TTFields probably had efficacy comparable to chemotherapy and bevacizumab.
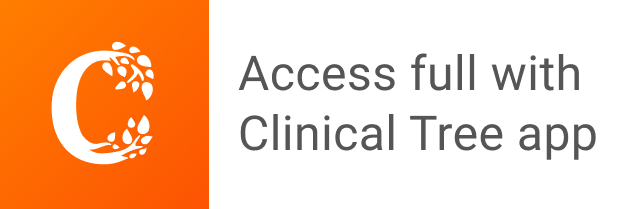