Chapter 12 Therapeutic ultrasound
INTRODUCTION
Ultrasound is almost certainly the most widely used of the ‘electrotherapy’ modalities in current clinical practice. In addition to its widespread use by physiotherapists (Pope et al 1995, ter Haar et al 1985), it is also commonly used by numerous therapists from other professional groups (e.g. osteopaths, chiropractors, sports therapists). Although widely considered as a form of electrotherapy, this is clearly not strictly the case in that ultrasound energy is a form of mechanical rather than electrical or electromagnetic energy (see Chapter 2), although the broader and in this case more accurate term ‘electrophysical agent’ would certainly encompass the modality comfortably.
Although therapeutic ultrasound has been used for over 40 years, its current use in the clinical environment has changed significantly over this period, and whereas in the past its use was primarily for its thermal effect, it is now more widely employed for its non-thermal effects, especially in relation to tissue repair and wound healing. There is a substantial volume of published evidence relating to the effects and use of ultrasound as a therapeutic modality, and this chapter aims to summarize this evidence.
The results of a survey carried out in Britain in 1985 (ter Haar et al 1985) showed that 20% of all physiotherapy treatments in NHS departments and 54% of all private treatments involved therapeutic ultrasound and the widely cited survey by Pope et al (1995) identified ultrasound as the most frequently employed modality (94%), with 64% of respondents reporting that they used the modality more than once a day (Fig. 12.1). In the 1985 survey, it was shown that there were large variations in the use of ultrasound including a range of intensities from 0.1 to 3.0W/cm2, giving a variation factor of 30 from the lowest to the highest applied intensity. The current application of ultrasound for fracture healing (see later) at even lower doses (0.03W/cm2) would take that to a factor of 100. This is a very substantial variation on just one factor that affects the output of the machine, and it is not surprising, therefore, that some research evidence is supportive of the modality whereas other publications are clearly not. If the effects of ‘electrotherapy’ interventions are both modality specific and dose specific (see Chapter 1), it is entirely predictable that this would be the case. Further identification of the machine and its dose parameters is clearly needed.
BEHAVIOUR OF ULTRASOUND
The physics of therapeutic ultrasound is covered in Chapter 2 and is not repeated in this section; however, it is important to consider some crucial behavioural characteristics before considering therapeutic effects and clinical use.
ULTRASOUND TRANSMISSION
All materials (tissues) present an impedance to the passage of sound waves. The specific impedance of a tissue will be determined by its density and elasticity. For the maximal transmission of energy from one medium to another, the impedance of the two media needs to be the same and thus the reflection that occurs at the interface will be minimized. Clearly, in the case of ultrasound passing from the generator to the body and then through the different tissue types, this cannot actually be achieved. The greater the difference in impedance at a boundary, the greater the reflection that will occur, and hence the smaller the amount of energy that will be transferred.
The coupling media used in this context include water, various oils, creams and gels. Ideally, the coupling medium should be sufficiently fluid to fill all available spaces; relatively viscous, so that it stays in place; have an impedance appropriate to the media it connects; and should allow transmission of ultrasound with minimal absorption, attenuation or disturbance (for extensive discussions regarding coupling media, see Casarotto et al 2004, Docker et al 1982, Klucinec et al 2000, Poltawski & Watson 2007a, Williams 1987). At the present time, the gel-based media are preferable to the oil- and cream-based media. Water is an effective medium and is a useful alternative but it fails to meet the above criteria in terms of its viscosity. A recent detailed study considering the effect of different coupling gels on ultrasound transmission demonstrated differences in transmission characteristics and absorption levels of commonly employed ultrasound gels, but there was no clinically significant difference between them (Poltawski & Watson 2007a).
PENETRATION AND ABSORPTION OF ULTRASOUND ENERGY
The absorption of ultrasound energy follows an exponential pattern, i.e. more energy is absorbed in the superficial tissues than in the deep tissues. For energy to have an effect, it must be absorbed. Transmission and attenuation of ultrasound energy are considered in detail in Chapter 2.
These values are not universally accepted (see Ward 1986) and ongoing research suggests that in the clinical environment they may be significantly lower. To achieve a particular ultrasound intensity at depth, account must be taken of the proportion of energy that has been absorbed by the tissues in the more superficial layers (Watson 2002).
As the penetration (or transmission) of ultrasound is not the same in each tissue type, it is clear that some tissues are capable of greater absorption of ultrasound than others. Generally, the tissues with the higher protein content will absorb ultrasound to a greater extent. This means that tissues such as blood and fat, which have a high water content and a low protein content, absorb little of the ultrasound energy, whereas those with a lower water content and a higher protein content will absorb ultrasound far more efficiently. It has been suggested that tissues can therefore be ranked according to their tissue absorption (Fig. 12.2). Although cartilage and bone are at the upper end of this scale, the problems associated with wave reflection at the tissue surface mean that a significant proportion of ultrasound energy striking the surface of either of these tissues is likely to be reflected. The best-absorbing tissues in terms of clinical practice are those with high collagen content: ligament, tendon, fascia, joint capsule and scar tissue (Frizzel & Dunn 1982, Nussbaum 1998, ter Haar 1999, Watson 2000, 2002).
The application of therapeutic ultrasound to tissues with a low energy-absorption capacity is less likely to be effective than the application of the energy into a more highly absorbing material. Recent evidence of the ineffectiveness of such an intervention can be found in Wilkin et al (2004), whereas application in tissue that is a better absorber will, as expected, result in a more effective intervention (Leung et al 2004a, Sparrow et al 2005).
EFFECTS OF ULTRASOUND
When it enters the body, ultrasound exerts an effect on the cells and tissues via two physical mechanisms: thermal and non-thermal. It is important that these mechanisms are understood, as some are stimulatory in their effect on the tissue repair process whereas others are potentially dangerous (for further details of the physical principles that underlie the behaviour of ultrasound, see Chapter 2).
THERMAL EFFECTS
When ultrasound travels through tissue a percentage of it is absorbed, and this leads to the generation of heat within that tissue. The amount of absorption depends upon the nature of the tissue, its degree of vascularization, and the frequency of the applied ultrasound. Tissues with a high protein content absorb ultrasound more readily than those with a higher fat content, and also the higher the ultrasound frequency, the greater the absorption rate. A biologically significant thermal effect can be achieved if the temperature of the tissue is raised to between 40 and 45°C for at least 5 minutes. Controlled heating can produce desirable effects (Lehmann & De Lateur 1982), which include pain relief, decrease in joint stiffness and increased local blood flow. The therapeutic benefits of tissue heating are well established (see Chapter 7 and 9) but ultrasound is relatively inefficient at generating sufficient thermal change in the tissues to achieve this therapeutic effect.
Historically, ultrasound has been widely employed for its thermal effects but it has been argued more recently that the ‘non-thermal’ effects of this energy form are more effective (Watson 2000, 2006a), and currently the majority of clinical applications focus on these. Gallo et al (2004) demonstrated that both continuous and pulsed ultrasound interventions generated measurable thermal changes in the tissues (muscle) but that, considering the temperature changes achieved, they would be expected to be of minimal therapeutic value. Garrett et al (2000) compared the heating effect of a pulsed shortwave treatment with an ultrasound treatment; the pulsed shortwave intervention was clearly more effective at achieving the required thermal change for therapeutic benefit. A patient treated with ultrasound in continuous mode at a relatively high dose will feel a temperature change (mainly in the skin, which is where thermal receptors are predominantly located) but this is not the same as achieving a clinically significant thermal change in deeper tissues.
A possible complication can occur when an ultrasound beam strikes bone or a metal prosthesis. Because of the great acoustic impedance difference between these structures and the surrounding soft tissues at least 30% of the incident energy will be reflected back through the soft tissue. This means that further energy is deposited as heat during the beam’s return journey. Therefore, heat rise in soft tissue could be higher when it is situated in front of a reflector. To complicate matters further, an interaction termed mode conversion occurs at the interface of the soft tissue and the reflector (e.g. bone or metal prosthesis). During mode conversion, a percentage of the reflected incident energy is converted from a longitudinal waveform into a transverse or shear waveform, which cannot propagate on the soft tissue side of the interface and is therefore absorbed rapidly, causing heat rise (and sometimes pain) at the bone-soft-tissue interface (periosteum). It is suggested that by maintaining a ‘moving’ treatment head approach and by using pulsed ultrasound, both of these effects will be minimized and will not constitute a clinical risk to the tissues. The greatest risk would be achieved with a high-dose, continuous ultrasound treatment using a stationary treatment applicator. The application of ultrasound over superficial bone or metal in the tissues is not contraindicated (see Chapter 21) although caution is advocated.
NON-THERMAL EFFECTS
There are many situations where ultrasound produces bioeffects and yet significant temperature change is not involved [e.g. low spatial-average temporal-average (SATA) intensity]. It is not strictly true to talk about ‘non-thermal’ mechanisms, in that the delivery and absorption of energy in the tissues will result in a temperature rise. The term ‘non-thermal’ in this context relates to the fact that there is no apparent thermal accumulation in the tissues (see Chapter 8) and is sometimes now referred to as a ‘microthermal’ effect. The term ‘non-thermal’ will be employed in the latter context throughout this section.
There is evidence indicating that non-thermal mechanisms play a primary role in producing a therapeutically significant effect: stimulation of tissue regeneration (Dyson et al 1968), soft-tissue repair (Dyson et al 1976, Watson 2006a), blood flow in chronically ischaemic tissues (Hogan et al 1982), protein synthesis (Webster et al 1978) and bone repair (Dyson & Brookes 1983, Malizos et al 2006).
The physical mechanisms thought to be involved in producing these non-thermal effects are one or more of the following: cavitation, acoustic streaming and standing waves (Baker et al 2001, ter Haar 1999, Williams 1987).
Cavitation
Ultrasound can cause the formation of micrometer-sized bubbles or cavities in gas-containing fluids. Depending on the pressure amplitude of the energy, the resultant bubbles can be either useful or dangerous. Low-pressure amplitudes result in the formation of bubbles that vibrate to a degree where reversible permeability changes are produced in cell membranes near to the cavitational event (Mortimer & Dyson 1988). Changes in cell permeability to various ions such as calcium can have a profound effect upon the activity of the cell (Sutherland & Rall 1968). High-pressure amplitudes can result in a more violent cavitational event (often called transient or collapse cavitation). During this event, the bubbles collapse during the positive pressure part of the cycle with such ferocity that pressures in excess of 1000MPa and temperatures in excess of 10000K are generated. This violent behaviour can lead to the formation of highly reactive free radicals. Although free radicals are produced by cells naturally (e.g. during cellular respiration), they are removed by free-radical scavengers. Production in excess of the natural free-radical scavenger system capacity could, however, be damaging. Avoidance of a standing-wave field and use of low intensities during therapy make it unlikely that transient cavitation will occur and there is no evidence that these effects are achieved in the tissues with therapeutic ultrasound when used appropriately. Some applications of ultrasound deliberately employ the unstable cavitation effect (high-intensity focused ultrasound; HIFU) but it is beyond the remit of this chapter (Wu 2006).
Acoustic streaming
This refers to the unidirectional movement of a fluid in an ultrasound field. High-velocity gradients develop next to boundaries between fluids and structures such as cells, bubbles and tissue fibres. Acoustic streaming can stimulate cell activity if it occurs at the boundary of the cell membrane and the surrounding fluid. The resultant viscous stress on the membrane, providing it is not too severe, can alter the membrane’s permeability and second-messenger activity (Dyson 1982, 1985). This could result in therapeutically advantageous changes such as increased protein synthesis (Webster et al 1978), increased secretion from mast cells (Fyfe & Chahl 1982), fibroblast mobility changes (Mummery 1978), increased uptake of the second-messenger calcium (Mortimer & Dyson 1988, Mummery 1978), and increased production of growth factors by macrophages (Young & Dyson 1990a). All these effects could account for the acceleration of repair following ultrasound therapy.
Standing waves
When an ultrasound wave hits the interface between two tissues of different acoustic impedances (e.g. bone and muscle), reflection of a percentage of the wave will occur. The reflected waves can interact with oncoming incident waves to form a standing-wave field in which the peaks of intensity (antinodes; see Chapter 2) of the waves are stationary and are separated by half a wavelength. Because the standing wave consists of two superimposed waves in addition to a travelling component, the peak intensities and pressures are higher than the normal incident wave. Between the antinodes, which are points of maximum and minimum pressure, are nodes, which are points of fixed pressure. Gas bubbles collect at the antinodes and cells (if in suspension) collect at the nodes (National Council on Radiation Protection (NCRP) 1983). Fixed cells, such as endothelial cells, which line the blood vessels, can be damaged by micro-streaming forces around bubbles if they are situated at the pressure antinodes. Erythrocytes can be lysed if they are swept through the arrays of bubblessituated at the pressure antinodes. Reversible blood cell stasis has been demonstrated, the cells forming bands half a wavelength apart centred on the pressure nodes (Dyson et al 1974). The increased pressure produced in standing-wave fields can cause transient cavitation and consequently the formation of free radicals (Nyborg 1977). It is therefore important to move the applicator continuously throughout treatment, and also use the lowest intensity required to cause an effect, to minimize the hazards involved in standing-wave field production (Dyson et al 1974).
The combination of stable cavitation and acoustic streaming is thought to be responsible for the cellular ‘upregulation’ that occurs during an ultrasound treatment. The therapeutic effects (see below) of this type of intervention arise as a consequence of this stimulated cellular activity and, strictly speaking, the ‘effects’ of ultrasound are at a cell membrane level (Fig. 12.3).
TISSUE REPAIR
Following injury, a number of cellular and chemical events occur in soft tissues. Although these events are explained in detail in Chapter 4, they aresummarized here in the context of ultrasound therapy.
UNDERLYING REPAIR PROCESS
For convenience, the whole repair process can be divided into several phases (Singer & Clark 1999, Watson 2006b), although it must be stated that these phases overlap considerably, lacking any distinct border between them. The three phases are:
Inflammation
This early, dynamic phase of repair is characterized initially by clot formation. Blood platelets are a major constituent of the blood clot and, in addition to their activities associated with clotting, platelets also contain numerous biologically active substances, including prostaglandins, and serotonin and platelet-derived growth factor (PDGF). These substances have a profound effect on the local environment of the wound and its subsequent repair (Singer & Clark 1999). Mast cells present another source of biologically active substances – or wound factors – which help orchestrate the early repair sequences.
Proliferation/granulation tissue formation
Wound contraction occurs during this phase of repair and can be defined as the process by which the size of a wound decreases by the centripetal movement of the whole thickness of surrounding skin (Peacock 1984). Myofibroblasts appear to play an important role in this process (Desmouliere et al 2005).
Remodelling
Remodelling can continue for many months or years after the proliferative phase of repair. During remodelling, granulation tissue is gradually replaced by a scar composed of relatively acellular and avascular tissue. As the repair matures, the composition of the extracellular matrix changes. Initially, it is composed of hyaluronic acid, fibronectin and collagens types I, III and V. The ratio of type I to III collagen changes during remodelling until type I is dominant. Scar tissue is a substitute for undamaged tissue. The rate at which wounds gain tensile strength is slow (Levenson et al 1965), and they are at only 20-25% of their maximum strength 3 weeks after injury. The increase in wound strength depends on two main factors: first, the rate of collagen deposition, remodelling and alignment, with the gradual formation of larger collagen bundles (Kischer & Shetlar 1974); second, alteration in the intermolecular cross-links (Bailey et al 1975). It will be shown later in this chapter that ultrasound can improve both the mechanical properties and functional capacity of the resulting scar tissue.
THE EFFECT OF ULTRASOUND ON THE INFLAMMATORY PHASE OF REPAIR
The inflammatory phase is extremely dynamic and numerous cell types (e.g. platelets, mast cells, macrophages and neutrophils) enter and leave the wound site. There is evidence to show that therapeutic ultrasound can interact with the above cells, influencing their activity and leading to the acceleration of repair (Fyfe & Chahl 1982, Maxwell 1992, Nussbaum 1997, ter Haar 1999, Watson, 2006a).
Acoustic streaming forces have been shown to produce changes in platelet membrane permeability leading to the release of serotonin (Williams 1974, Williams et al 1976). In addition to serotonin, platelets contain wound factors essential for successful repair (Ginsberg 1981). If streaming can stimulate the release of serotonin, it is proposed that it may also influence the release of these other factors.
One of the major chemicals that modifies the wound environment at this time after injury is histamine. The mast cell is the major source of this factor, which is normally released by a process known as mast cell degranulation. In this process, the membrane of the cell, in response to increased levels of intracellular calcium (Yurt 1981), ruptures and releases histamine and other products into the wound site. It has been shown that a single treatment of therapeutic ultrasound, if given soon after injury (i.e. during the early inflammatory phase), can stimulate mast cells to degranulate, thereby releasing histamine into the surrounding tissues (Fyfe & Chahl 1982, Hashish 1986). It is possible that ultrasound is stimulating the mast cell to degranulate by increasing its permeability to calcium. Increased calcium ion permeability has been demonstrated by a number of researchers. Calcium ions can act as intracellular messengers; when their distribution and concentration change in response to environmental modifications of the plasma membrane they act as an intracellular signal for the appropriate metabolic response (Leung et al 2004a, Mortimer & Dyson 1988, Nussbaum 1997).
Reversible membrane permeability changes to calcium have been demonstrated using therapeutic levels of ultrasound (Dinno et al 1989, Mortimer & Dyson 1988, Mummery 1978). The fact that this effect can be suppressed by irradiation under pressure suggests that cavitation is the physical mechanism responsible. Changes in permeability to other ions such as potassium have also been demonstrated (Chapman et al 1979). Work by Dinno et al (1989) demonstrated that ultrasound can modify the electrophysiological properties of skin; this study reported an ultrasound-induced reduction in the sodium/potassium ATPase pump activity. A decrease in pump activity, if it occurs in neuronal plasma membranes, may inhibit the transduction of noxious stimuli and subsequent neural transmission, which may account in part for the pain relief that is often experienced following clinical exposure to therapeutic ultrasound, although as a modality, ultrasound is not often applied with the primary intention of achieving pain relief, and is considered to be a secondary benefit rather than of primary importance. It should be noted, however, that the mechanism of ultrasound-induced pain relief is still not fully understood and that it may be attributed, in part at least, to placebo effects.
As identified above, the evidence is clear: therapeutic ultrasound can alter membrane permeability to various ions. The ability to affect calcium transport through cell membranes is of considerable clinical significance as calcium, in its role as an intracellular or second messenger, can have a profound effect on cell activity, for instance by increasing synthesis and secretion of wound factors by cells involved in the healing process. This has been shown to occur in macrophages in response to therapeutic levels of ultrasound (Young & Dyson 1990a) and these are one of the key cells in the wound-healing system, being a source of numerous wound factors. This in-vitro study demonstrated that the ultrasound-induced change in wound factor secretion is frequency dependent. Ultrasound at an intensity of 0.5W/cm2 (SATA) and a frequency of 0.75MHz appeared to be most effective in encouraging the immediate release of factors already present in the cell cytoplasm, whereas the higher frequency 3.0MHz appeared to be most effective in stimulating the production of new factors, which were then released some time later by the cells’ normal secretory processes. Therefore, there appeared to be a delayed effect when treating with the higher frequency; however, the resulting liberated factors, when compared with those liberated using 0.75MHz, were more potent in their effect on the stimulation of fibroblast population growth. One possible reason why these two frequencies induce different effects relates to the physical mechanisms involved. At each frequency the peak pressure generated by the ultrasound was that necessary for cavitation to occur (Williams 1987). Cavitation is more likely to occur at the lower frequency, whereas heating is more likely to occur at the higher one. Therefore, the differing proportions of non-thermal to thermal mechanisms present in each of the two treatments may explain the difference seen in the resulting biological effects.
Hart (1993) also found that following the in vitro exposure of macrophages to ultrasound, a wound factor was released into the surrounding medium that was mitogenic for fibroblasts.
It has often been thought that ultrasound is an anti-inflammatory agent (Reid 1981, Snow & Johnson 1988). When viewed from a clinical standpoint – that is, rapid resolution of oedema (El Hag et al 1985) – this conclusion is understandable. However, research has shown that ultrasound is not anti-inflammatory in its action (Goddard et al 1983, Watson 2006a); rather, it encourages oedema formation to occur more rapidly (Fyfe & Chahl 1985, Hustler et al 1978) and then to subside more rapidly than control sham-irradiated groups, so accelerating the whole process and driving the wound into the proliferative phase of repair sooner.
Further confirmation of this has been shown experimentally in acute surgical wounds (Young & Dyson 1990b). In this study, full-thickness excised skin lesions in rats were exposed to therapeutic ultrasound (0.1W/cm2 SATA, 0.75MHz or 3.0MHz) daily for 7 days (5 minutes per day per wound). By 5 days after injury, the ultrasound-treated groups had significantly fewer inflammatory cells in the wound bed and more extensive granulation tissue than the sham-irradiated controls. Also, the alignment of the fibroblasts – parallel to the wound surface – in the wound beds of the ultrasound-treated groups was indicative of a more advanced stage of tissue organization than the random alignment of fibroblasts seen in the sham-irradiated control wounds. The results obtained suggest that there had been an acceleration of the wounds through the inflammatory phase repair in response to ultrasound therapy. It was also noted that there were no abnormalities such as hypertrophy of the wound tissue seen in response to ultrasound therapy.
Therefore, ultrasound therapy appears to accelerate the process without the risk of interfering with the control mechanisms that limit the development of repair materials. By increasing the activity of these cells, the overall influence of therapeutic ultrasound is certainly pro- rather than anti-inflammatory. The benefit of this mode of action is not to ‘increase’ the inflammatory response as such (although, if applied with too great an intensity at this stage, it is a possible outcome; Ciccone et al 1991), but rather to act as an ‘inflammatory optimizer’. The inflammatory response is essential to the effective repair of tissue, and the more efficiently the process can complete, the more effectively the tissue can progress to the next (proliferative) phase.
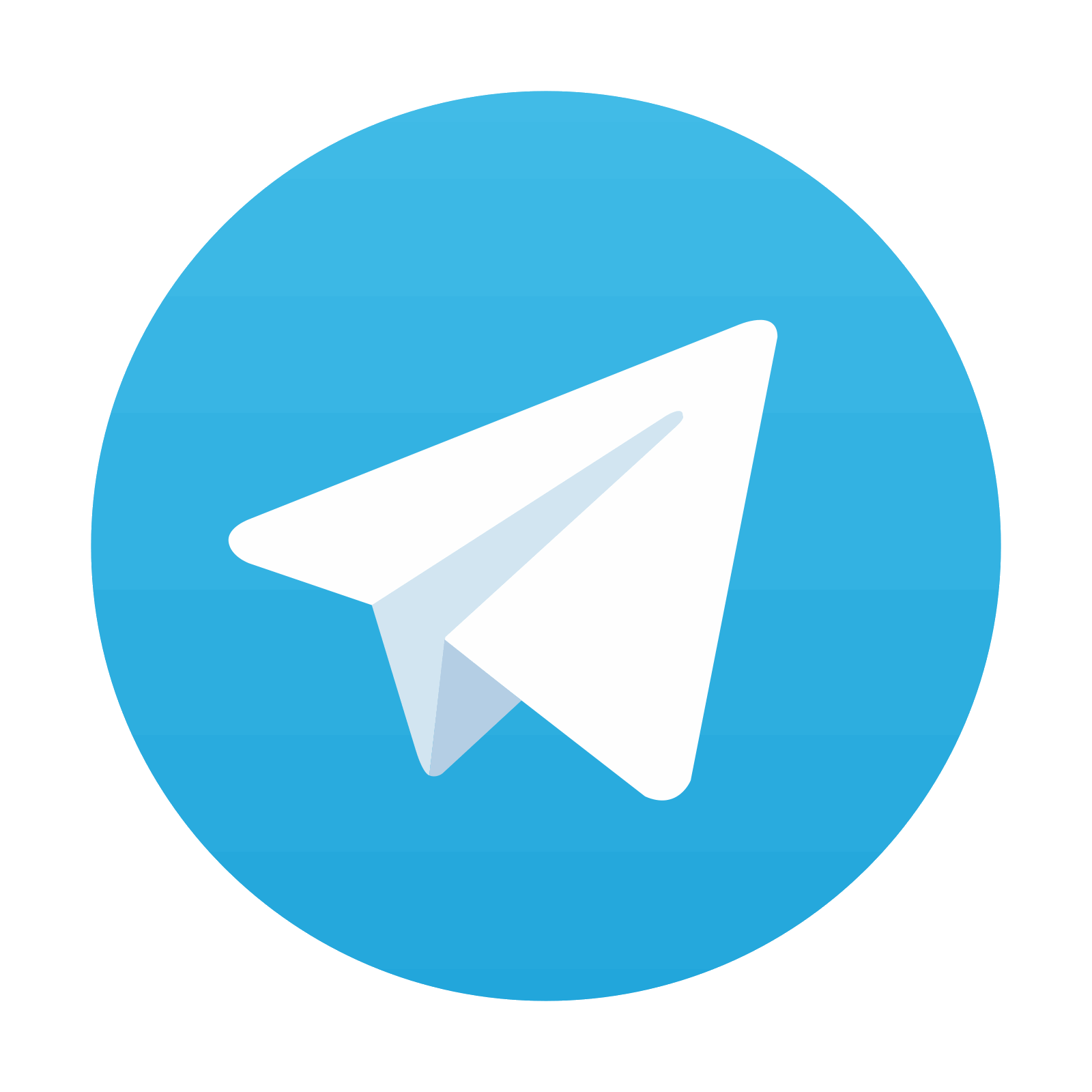
Stay updated, free articles. Join our Telegram channel
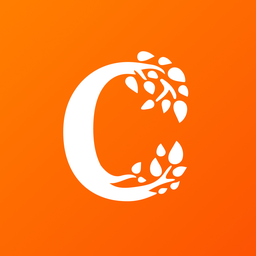
Full access? Get Clinical Tree
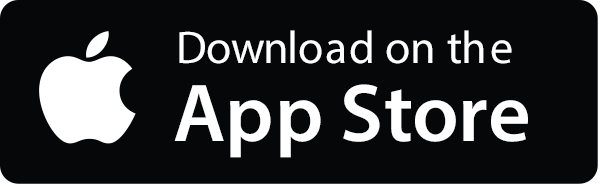
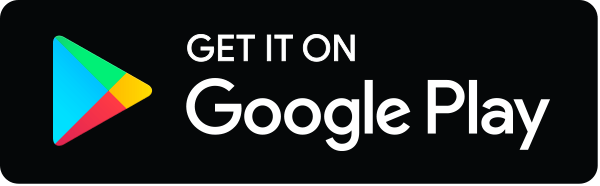