Fig. 18.1
NanoTechnology elements for NanoNeuroscience. (a) From graphene to single wall carbon nanotubes. (b) From graphene to multiple wall carbon nanotubes. (c–d) Axial and radial nanowires based heterojunctions, e.g., p-n-p, n-p-n, p-i-n
Different technological fields are witnessing huge promises from CNTs based on their unique properties, such as being used in probes and interconnects as conductive composites, nanometer-sized semiconductor devices, field emission displays and radiation sources, hydrogen storage media, sensors, energy storage and energy conversion devices (Sharma and Ahuja 2008). Functionalization of the CNTs surface was performed using many different approaches. CNTs have been proposed either by themselves or as components for biosensors (Wenrong et al. 2010), ion channel blockers (Park et al. 2003), biocatalysts (Feng and Ji 2011), photo-thermal probes in cancer therapy (Moon et al. 2009), nanovectors (Klumpp et al. 2006) and imaging applications (Kam et al. 2005a; Wu et al. 2005; Klumpp et al. 2006).
Imaging applications of CNTs to living cells and neural tissues bring promising advantages for neurobiological applications based on the optical properties of nanotubes. Due to the high photostability of SWCNTs photoluminescence, a longer excitation time is attainable in CNTs at higher laser fluency compared to quantum dots or organic fluorophores. An attenuated absorption, combined with autofluorescence and scattering characteristics makes opaque tissue visible in the range of 700–1,400 nm allowing imaging of the whole blood or thick tissue (Heller et al. 2006). This is mainly because the fluorescence profiles of many semiconducting nanotubes overlap with the wavelength range. The imaging SWCNTs in tissue sections and the nanotubes concentration measured in blood is based on nanotube fluorescence (Cherukuri et al. 2006). On the other hand, CNTs can be detected due to their large resonance-enhanced Raman scattering (Heller et al. 2005). In addition, SWCNTs show confined heating upon near infrared (NIR) absorption because SWCNTs absorb strongly in NIR wavelengths range (Endo et al. 1990). SWCNTs released DNA due to exposure at NIR radiation permits its translocation into the cell nucleus (Kam et al. 2005b). Cell death demonstrated in the same study was due to the fact that SWCNTs were causing a local heating upon exposure at NIR radiation, triggering the killing of the cells.
The transport of nanotubes into cells is of fundamental importance for the biomedical applications mentioned. As yet, the way in which CNTs enter cells is still under hot debate, generating both controversy and confusion about the mechanism by which they enter cells. Work by Bianco and colleagues (Bianco et al. 2011) suggests that ammonium-functionalized SWCNTs and MWCNTs are formed via a passive, endocytosis-independent mechanism (Pantarotto et al. 2004b), while Dai and colleagues (Dai et al. 2001) conclude that the mechanism of the acid-functionalized SWCNTs entering the cell involves an endocytosis pathway (Kam et al. 2004). Alternate mechanism is proposed for MWCNTs, which cannot use the endocytosis pathway due to their size. The mechanism proposed for MWCNTs takes into consideration the flipping of lipid molecules of the membrane to allow CNTs to enter the cell (Kam et al. 2004; Lopez et al. 2004; Lu et al. 2004; Pantarotto et al. 2004a, b; Cai et al. 2005; Kam and Dai 2005).
The communication between cell and nanotube is ruled by the type of coating on the nanotube surface. By transferring CNTs into cells, proteins are adsorbed to the nanotube surface, coating the nanotube with serum containing proteins, such as albumin and fibronectin. However, it was suggested that CNTs transfer into cells have a natural switching mechanism of lipids in the membrane (Lopez et al. 2004; Pantarotto et al. 2004a) and do not have an endocytotic pathway for the MWCNTs that are 200 nm in length with 10 nm radius. Open questions are what happens to CNTs once they have entered the cell and also when or how CNTs would be exocytosed by the cells (Sakhtianchi et al. 2013). The possibility for nanotubes to be subsequently expelled from the cell seems advantageous for most biological applications; although, as yet, this has not been reported in the literature. There is still much work necessary to understand the CNTs cellular transport to be able to control the CNTs placement inside cells (Endo et al. 1990).
The therapeutic effect of drugs is steadily improving through the development of new delivery vehicles. CNTs have a higher surface area to volume ratio than spheres have, giving nanotubes the potential to be conjugated with more functional agents (Wu et al. 2005) and to accommodate large loads of therapeutic agents (Kam et al. 2005a). Previously, these vehicles included viral vectors, liposomes, cationic lipids, polymers, and nanoparticles. Although nonviral vehicles have versatility of shape, size, and materials, one issue of concern is the poor penetration of some therapeutic agents into cells. CNTs are readily internalized by cells, and after surface modification, they exhibit low cytotoxicity over the period of a few days (Kam et al. 2004, 2005a; Lu et al. 2004; Pantarotto et al. 2004a, b; Cai et al. 2005; Kam and Dai 2005; Wu et al. 2005).
18.2.2 Nanowire Junctions
The range of length scale in biology varies by several orders of magnitudes—from nanometer sized nucleic or amino acids to several centimeters for organs and neuronal circuits. There is a need for interfaces with nanoscale spatial resolution in order to investigate processes at the subcellular level. Besides carbon nanotubes, these interfaces can be achieved through the use of other nanostructures, such as semiconductor nanowires having dimensions as small as a protein molecule. CNTs and NWs represent the building blocks for nanoscale electronics (McEuen et al. 2002; Lieber 2003). The critical feature sizes (atomic scale) of these building blocks can be well controlled during synthesis, in contrast with the nanostructures fabricated by the “top-down” process. Even for isolated CNTs transistors that have shown exceptional properties (Javey et al. 2003), large scale integration challenges remain due to difficulties in preparing pure semiconductor nanotubes. The issues faced by CNTs could be overcome by nanowires because of the reproducible control over size and electronic properties that current growth methods enable (Cui et al. 2001b, 2003; Cui and Lieber 2001; Wu et al. 2004). A good class of NWs has been developed, ranging from NWs based on classic semiconductors, such as silicon NWs (Chen et al. 2006; Goncher et al. 2006; Yajie et al. 2008), GaP (Dujavova-Laurencikova et al. 2013), GaN (Lee et al. 2007), CdS and ZnS (Barrelet et al. 2003), heterostructures as Ge-Si (Xiang et al. 2006a, b), InAs-InP (Jiang et al. 2007), oxide nanowires MgO (Yin et al. 2002), Cu2O (Jiang et al. 2002), SiO2 (Yu et al. 1998; Liu et al. 2001; Zheng et al. 2002), Ga2O3 (Wu et al. 2000; Sharma and Sunkara 2002), Al2O3 (Valcarcel et al. 1998; Xiao et al. 2002), In2O3 (Li et al. 2003), SnO2 (Dai et al. 2001), MnO2 (Wang and Li 2002), Sb2O3 (Guo et al. 2000), TiO2 (Seraji et al. 2000; Miao et al. 2002), ZnO (Tian et al. 2003; Vayssieres 2003), LiNiO2 (Zhou et al. 2002), and others.
The field-effect transistors (FETs) configuration of semiconductor NWs is one of the most appropriate detection schemes for biological events (Cui and Lieber 2001; Cui et al. 2001a, 2003; Zheng et al. 2004; Xiang et al. 2006b). An illustration of a FET schematic is depicted in Fig. 18.2. The binding to the dielectric gate of a polar/charged species appears analogous to applying a voltage to a gate electrode. For example, accumulation of positive carriers (holes) together with an increase/variation in device conductance can be generated by binding a protein with a negative charge to the surface of a p-type FET. Silicon based NWs (or other types of semiconductors) also may function as FET devices (Cui and Lieber 2001; Cui et al. 2003; Lieber 2003; Zheng et al. 2004; Li et al. 2006; Xiang et al. 2006b). One-dimensional morphology of NWs is the main feature that determines overcoming sensitivity limitations for planar FET devices. Thus, a more substantial change in device conductance for the NWs versus a planar FET will take place if any analyte binding event will happen (this event leads to accumulation or depletion of carriers).


Fig. 18.2
Schematics of a field-effect transistor (FET) using as a gate channel a NW or a CNT. Its main elements are: Source electrode (S), Drain electrode (D), Bottom/back gate electrode (BG), Gate dielectric (SiO2 or high-κ dielectric). An analyte binding to the FET channel is suggested
18.2.3 Nanowires-Based Neural Devices
One of the most powerful and versatile platforms for building functional interfaces to biological systems (including neurons) is based on NWs devices. NWs are highly local probes for neuronal projections because individual NWs devices are becoming optimal for interfacing with neurons, mainly due to the contact length along the axon, or the dendrite projection crossing a NW, being just about 20 nm. Compared to micro-fabricated electrodes and planar FETs, the active junction area for NWs devices is orders of magnitude smaller.
An important advantage when compared to other electrophysiological methods is the small hybrid junction that is quite similar to natural synapses. This small size creates advantages such as: (a) spatially resolved signal detection without complicated averaging of the extracellular potential, which changes over a large portion of a given neuron, and (b) integration of the axon’s elements together with the dendrite projections from a single cell. The stimulation of neuronal activity through NW/axon junctions is also achievable using NWs devices. Somatic action potential spikes detected with intracellular electrodes are generated by applying excitatory sequences of biphasic pulses to the NWs of NW/axon junctions (Patolsky et al. 2007).
Additionally, a NW-based FET device array can be designed to promote neuron growth (Patolsky et al. 2007). Thus, interfacing ensembles of NWs inputs and outputs to different neuron ensembles enables the implementation of stimulation, inhibition, or reversibly blocking signal propagation through specific pathways allowing the signal flow to be simultaneously mapped throughout the network. Besides single or multiple arrays of NWs-based FET devices used for investigating neuronal activity, the NWs were also used to design and build NWs-based electrodes for neural recordings in the brain.
The potential to revolutionize neuroscience research and clinical therapy (Benabid 2007; Kipke et al. 2008; Vaadia and Birbaumer 2009; Suyatin et al. 2013) is represented by implantable neural interfaces (Rutten 2002; Fromherz 2003; Cogan 2008). However, the recorded neurons and tissue reactions that encapsulate and insulate the implant are still presenting instability results (Schouenborg 2011). The nanostructured electrodes are considered as a promising alternative to conventional neuronal interfaces because the recording properties depend mainly on electrode surface properties and tissue reactions to the surface (Kotov et al. 2009; Timko et al. 2010; Dvir et al. 2011; Suyatin et al. 2013). Nanostructured electrodes provide additional advantages such as improved electrical properties (Keefer et al. 2008; Cellot et al. 2009; Martin et al. 2010; Ansaldo et al. 2011; Duan et al. 2012), a shorter cell-to-electrode distance (Tian et al. 2010; Duan et al. 2012; Xie et al. 2012), and better spatial resolution. They also have a potential for less tissue damage (Almquist and Melosh 2010; Martin et al. 2010; Tian et al. 2010; Duan et al. 2012), better biocompatibility (Hallstrom et al. 2007; Kim et al. 2007; Martin et al. 2010; Berthing et al. 2011) and new functionalities, such as selective guidance of neuronal fibers (Hallstrom et al. 2009). Importantly, recordings of cell signals with different nanowire-based electrodes have been achieved in vitro (Kotov et al. 2009; Tian et al. 2010; Timko et al. 2010; Brueggemann et al. 2011; Dvir et al. 2011; Duan et al. 2012; Robinson et al. 2012; Xie et al. 2012), thus demonstrating that epitaxially grown wires of small diameter may provide a minimally invasive tissue penetration (Kawano et al. 2010; Takei et al. 2010; Tian et al. 2010; Duan et al. 2012; Xie et al. 2012). Until now, using mainly carbon nanotubes without structural feature control and in combination with rather big surfaces, has shown improved cell survival for neuronal interfaces (Hallstrom et al. 2007) and improved cell adhesion with focal adhesions forming specifically on the nanowires epitaxially grown, e.g., gallium phosphide (GaP) NWs have beneficial properties (Prinz et al. 2008). Compared to other material NWs, GaP NWs can be synthesized with very little tapering and exceptional control over their position and geometry, and with a high aspect ratio (the ratio between their length and their diameter) is over 50 (Suyatin et al. 2009). Also, the design and fabrication of a first generation of GaP NW-based electrode with a controllable nanomorphology was reported (Suyatin et al. 2013). The first functional testing in vivo of a NWs based device was performed during acute recordings in the rat cerebral cortex, where the NWs were used as a backbone for metal nanostructured electrode with a three-dimensional (3D) structure. This electrode design opened the development of a new model system, with the prospect of enabling a more reliable tissue anchoring as well as a more intimate contact between the electrode and the neurons (Xie et al. 2010). Further research on the functionality of nanostructure-based neuronal interfaces in vivo is providing better electrode-cell electrical coupling (Hai et al. 2010; Robinson et al. 2012; Xie et al. 2012).
18.3 Carbon Nanotubes in NeuroNanoTechnology
Carbon nanotubes have a multitude of useful properties (electrical, mechanical and chemical) that make them very promising materials for applications in neuroscience. The ease with which carbon nanotubes can be patterned permits use for studying the organization of neural networks while the electrical conductivity of nanotubes can provide a vital mechanism to monitor or stimulate the neurons. Carbon nanotubes can interact with neurons and affect neuronal function, especially at the level of ion channels (Malarkey and Parpura 2007, 2010). Both SWCNTs and MWCNTs have been increasingly used as “scaffolds for neuronal growth”. Recently, CNTs have been used in neural stem cell growth and differentiation research. Also, CNTs have been used as interface materials with neurons, where they can deliver electrical stimulation to these cells and detect electrical activity. Here we mention just few applications of the CNTs:
18.3.1 Interfacing Cultured Neurons with Carbon Nanotubes
To demonstrate induced neuronal signaling by electrical simulation involving single-wall carbon nanotubes (SWCNTs), Mazzatenta and colleagues developed an integrated SWCNTs neuronal system (Mazzatenta et al. 2007) showing that hippocampal cells can be grown on pure SWCNTs substrates. These experimental results point to the fact that SWCNTs can be directly used to stimulate brain circuit activity. This result may have an impact in the future developments and architectural design of microsystems for neural prosthetics (Mazzatenta et al. 2007).
18.3.2 Intracellular Neural Recording with Pure Carbon Nanotubes Probes
A novel millimeter-long electrode can be used to produce extracellular and intracellular recordings from vertebrate neurons in vitro and in vivo experiments, when it is terminated with a tip fabricated from self-entangled pure CNTs with sub-micron dimensions (Yoon et al. 2013). Assembling intracellular electrodes from CNTs using self-entangled CNTs fabrication technology is opening the way to “harness” nanotechnology for neuroscience applications.
18.3.3 Analog Neuromorphic Modules Based on Carbon Nanotube Synapses
Shen et al. (2013) recently reported an analog neuromorphic module consisting of an integrate-and-fire circuit and p-type carbon nanotubes synapses. The CNTs synapse resembles a FET structure using as its gate an aluminum oxide dielectric layer implanted with indium ions and as its channel a random CNTs network. The electrons are attracted into the defect sites of the gate aluminum oxide layer by a positive voltage pulse applied to the gate, followed by a gradual release of the trapped electrons after the pulse is removed. Thus, the electrons induce a dynamic postsynaptic current in the CNTs channel by modifying the holes concentration. The excitatory or inhibitory postsynaptic currents generated by multiple input pulses via excitatory or inhibitory CNTs synapses, flow toward an integrate-and-fire circuit that triggers output pulses. Further, the analysis of the dynamic transfer functions between the input and output pulses of the neuromorphic module can be performed. An emulation of biological neural networks and their functions could be implemented by scaling up such a neuromorphic module.
18.3.4 Nanotechnology and Nanocomputing
The last decade witnessed an increasing use of artificial intelligence tools in nanotechnology research (Sacha and Varona 2013). Convergence of the two sciences, nanocomputing and nanotechnology, has the potential to reshape current research directions and technological developments in virtually all sciences. Thus, nanocomputing hardware development can boost the field of artificial-intelligence-based applications. Combining nanotechnology and nanocomputing has also shown great potential of hybrid technologies (i.e., nano-device and biological components) in neuroscience, in bioengineering, which combines new data representations and computer architectures, and in a large variety of related disciplines.
18.4 Role of Carbon Nanotubes in Deciphering Cortical Microcircuit Function
The tools used to study the functions of the neocortex need to operate at the nano-level, i.e. the same scale as brain functions operate. Nanoscience together with nanotechnology bring together an arsenal of unique methods to examine the complexity of brain function by allowing concurrent recording of thousands of neurons and manipulating the activity of millions of cells. One major reason why we are interested in examining them relates to the fact that they are ideal elements to uncover the micro-connectivity between cortical neurons intra-layer and within cortical minicolumns (Wade and Katzman 1975; Gandhi et al. 2010; Qiu et al. 2010; Choi et al. 2012; Jiang et al. 2013). Huge effort is now being devoted to the decoding of specific neural interactions and circuits, a goal that has emerged as the Brain Activity Mapping Project (Alivisatos et al. 2013). Several examples of the rich synergy between nanotechnology and neuroscience are given as follows:
18.4.1 Multi-electrode Array Technologies for Brain Circuits
The “substrate-integrated” microelectrode array (MEA) is the finest approach to study brain circuitry and connectivity, neurophysiology, or pathology, both in vivo and in vitro. MEAs add real-time versatility to long-term recording of neurophysiological activity in the extra-cellular micro-environment along with biochemical fluctuations, while being minimally invasive (Wise 2007; Chang-Hsiao et al. 2010; Amaral et al. 2013). The micro-anatomy of a neural ensemble and its synaptic interconnectivity, excitability, and plasticity may be better monitored by MEAs.
18.4.1.1 Carbon Nanotube MEAs
Suzuki et al. (2013) developed MEA chips of planar CNTs that can measure both the electrophysiological responses (such as action potentials and field postsynaptic potentials) and the release of dopamine neurotransmitter. These CNTs-MEA chips have been fabricated directly on the microelectrode surfaces by electroplating an indium-tin oxide (Fig. 18.3a). Chronoamperometric measurements based on such CNTs-MEA chips detected dopamine concentration at nanomolar level with high temporal resolution and a 100-fold better signal to noise ratio. MEA chips may be useful for various applications such as drug screening and toxicity, in vitro stem cell differentiation, synaptic plasticity, or pathogenic processes associated with stroke, epilepsy, Alzheimer’s disease and other neurodegenerative conditions.


Fig. 18.3
Recording neural activity with MEA based on MWCNTs. (a) Schematic of multi electrode array based MWCNTs. (Left) cross-sectional view right. (Right) top view of the electrode scheme. (b) Schematic view of recording system. (c) Extracellular signal with large signal-to-noise ratio was obtained from lateral giant nerve fiber of an American crayfish using MEA based on MWCNTs and treated with steam plasma, compared with MWCNTs as grown (With permission from Chen et al. 2010)
18.4.1.2 Multi-walled Carbon Nanotube MEAs
MEAs using MWCNTs have the advantage of using a small size microelectrode with increased impedance and decreased charge-transfer capability (Gacem et al. 2013). To decrease impedance, the effective surface area for recording of the electrode needs to be increased. With a steam-plasma treatment the surface of MWCNTs becomes converted from super-hydrophobic to super-hydrophilic. This hydrophilic property is attributed to the OH bonding on the surface of MWCNTs. This electrode type allows the separation of neural signals with their distinct shapes for long-term recordings and improved recording performance.
Long-term recordings of modular features of the brain may be applied by this MEA type. In extracellular recording, action potentials were recorded with the treated MWCNTs; the signal-to-noise ratio (SNR) was up to 40 dB when used to record neural signals of a lateral giant cell from an American crayfish (Fig. 18.3b). Figure 18.3c shows the extracellular signal with large SNR obtained from the nerve fiber using MEA based on MWCNTs treated with steam plasma, compared with MWCNTs as grown.
18.4.1.3 Multiplexed High Density MEAs
Recent neural probes based on silicon (Du et al. 2011) have employed nanofabricated, high-density electrical leads that can read out multichannel data. MEA uses “application-specific integrated circuit” (ASIC) which intensifies signals, multiplexing functions and band-pass filtering. A multiplex high density device with fully integrated, low noise, 64-channel system can perform high spatial resolution extracellular measurements and weighs just 330 mg (Du et al. 2011). These on-chip multiplexers allow recordings with “substantially fewer external wires than the number of input channels”. The combination of ASICs and nanofabricated probes that is both “minimally invasive and highly scalable” (Du et al. 2011) was employed for carrying out large-scale, high-density electrophysiology in small animals.
Similarly, Viventi et al. (2011) integrated “ultrathin and flexible silicon nanomembrane transistors” into a MEA, enabling “dense arrays” of thousands of amplified and multiplexed sensors to be connected with fewer wires. This system was employed to record in cat the spatial properties of brain activity in vivo, including patterns of activity such as sleep spindles, single-trial visual evoked responses, or electrographic seizures. Such developments promise to provide diagnostic and therapeutic brain-machine interface devices.
The MEA system containing a 64-site array (Fig. 18.4a) was implanted into the mouse hippocampus (Fig. 18.4a, b). The animal was then connected to a flexible 12 wire tethered cable and allowed to explore a 30 cm × 50 cm enclosure during acquisition of neurophysiological data. Current source density (CSD) analysis of local field potential signals with a vertical resolution of ~28 mm revealed relatively uniform theta oscillations in layers between the CA1 and dentate gyrus (Fig. 18.4b). However, marked shifts in amplitude and phase were observed on sites around the suprapyramidal and infrapyramidal blades of the dentate gyrus. Theta phase dependent oscillatory firing of hippocampal neurons were observed during exploratory behavior (Fig. 18.4b). As expected, hippocampal neurons were found to be preferentially active in the vicinity of the negative peak of the theta potential.


Fig. 18.4
Recording with nanofabricated multi-electrode-arrays in awake behaving mice. (a) Nanofabrication of 64 channels neural probes. (b) Neuron recordings with nanofabricated probes in awake behaving mice. (1) Nissl-stained brain section overlaid with a schematic of the probe at its stereotaxically implanted location. Each silicon shaft is 60 mm wide. Scale bar, 200 mm. (2) Current source density analysis of local field potentials across the hippocampus with a vertical resolution of ~28 mm. Measurements correspond to the rightmost shaft, which is co-localized with both the supra- and infra-pyramidal blades of the dentate gyrus (DG). The data were gathered during home cage exploratory behavior. The CSD is normalized to ±1. Scale bar, 100 ms. (3) Waveforms of two putative single units recorded from this probe across all sites on their entire respective shafts, with histograms showing theta phase locking of spikes. Dashed ellipses indicate the sites exhibiting the highest extracellular action potentials for these units. Measured theta oscillations on top of the histograms are for reference. Theta oscillations were measured from the upper rightmost electrode near the CA1 pyramidal cell layer (Du et al. 2011)
Figure 18.5 displays a sample of spontaneous firing activity in the ventral posteromedial thalamic nucleus of an anesthetized mouse, recorded by Du and colleagues (Du et al. 2011). The data shows the majority of functional (63/64) electrodes in the 1.3 mm long MEA have spiking activity, indicating that more than one electrode often records from the same neuron. The close electrode spacing is thus well suited for high-density electrophysiology with extensive coverage of extracellular fields along the length of the array. High spatial resolution extracellular measurements with a fully integrated, low noise 64-channel system enabled reliable spike detection with peak amplitudes as low as 40–50 μV (Du et al. 2011).


Fig. 18.5
Parallel recording capabilities of the multiplexed neurophysiological system. Traces are filtered from 0.3–5 kHz to highlight spiking activity, and are plotted near their corresponding location on the shaft of the probe displayed on the left (for each horizontal left-right pair of pads, the trace for the left-hand pad appears just above the trace for the right-hand probe). Measurements were made in the mouse thalamus with a probe with post-plating impedances of 0.6 MΩ ± 0.4 MΩ (mean ± 1 s.d.). The majority of the 64 sites report action potentials (Du et al. 2011)
18.4.1.4 Nanowire-Based Electrode for Acute Neural Recordings in the Brain
A new kind of electrode is based on “structurally controlled nanowires,” for neurophysiological measurements in vivo (Suyatin et al. 2009). This electrode (Fig. 18.6) has a sensing part made of a thin metal layer deposited on epitaxially grown GaP nanowires. Suyatin and colleagues realized the first functional NWs-based electrode (Suyatin et al. 2009). The team also has successfully tested the electrode by in vivo recordings in the cortex of rat in multiple brain implantations. This kind of electrode with a controllable geometry of the nanowires can be further used for the investigation of many in vivo functional properties in nanostructured neuronal interfaces.


Fig. 18.6
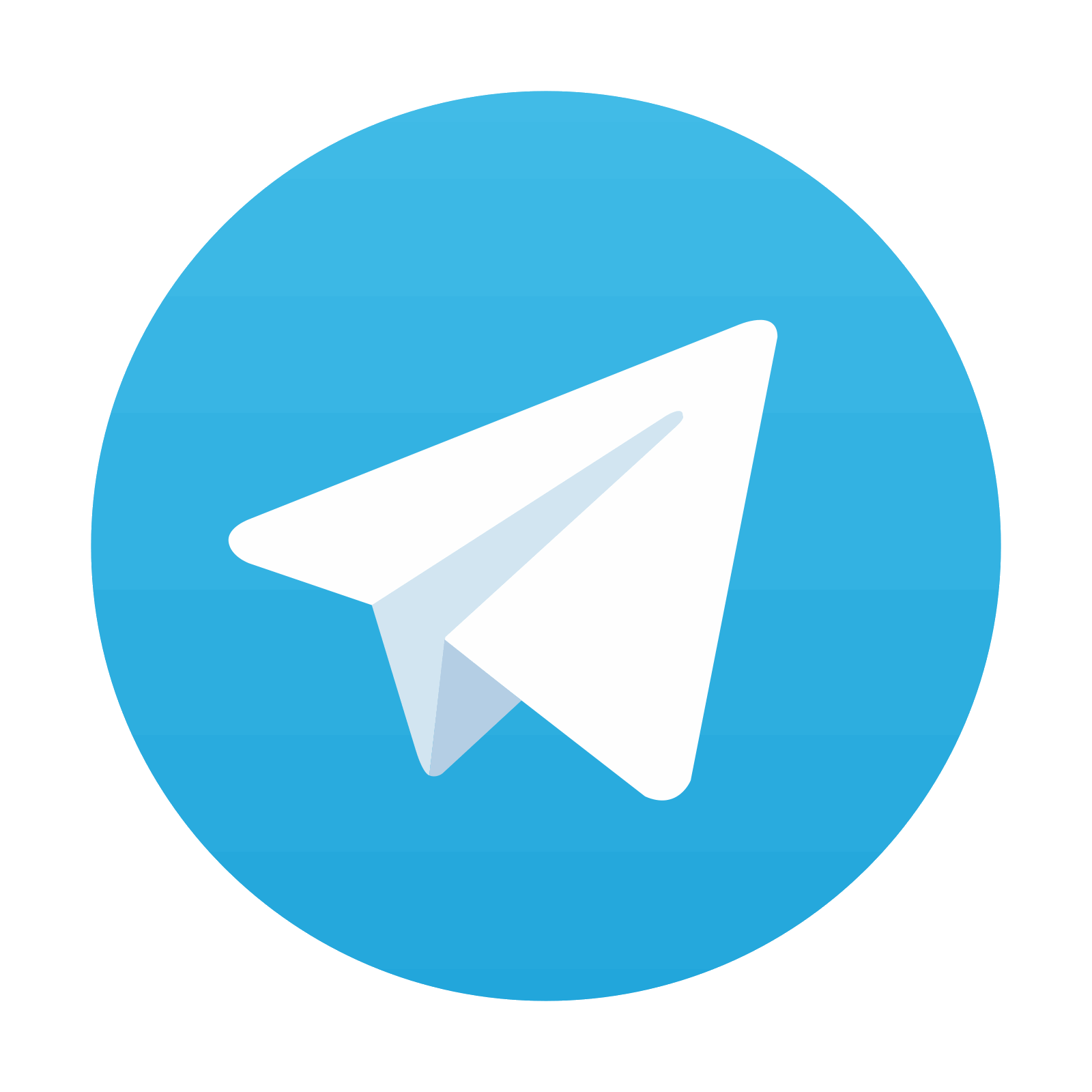
Nanowire-based electrode for acute in vivo neuronal signal recordings. (a) Scanning electron microscope (SEM) image of the nanowire-based electrode tip. (b) SEM image of the nanowire-based sensing region made with an array of freestanding vertical gallium phosphide nanowires covered with hafnium oxide and metal film. (c) Layout for the nanowire-based electrode. (d) Schematic for the nanowire geometry and the electrode layered structure (With permission from Suyatin et al. 2013)
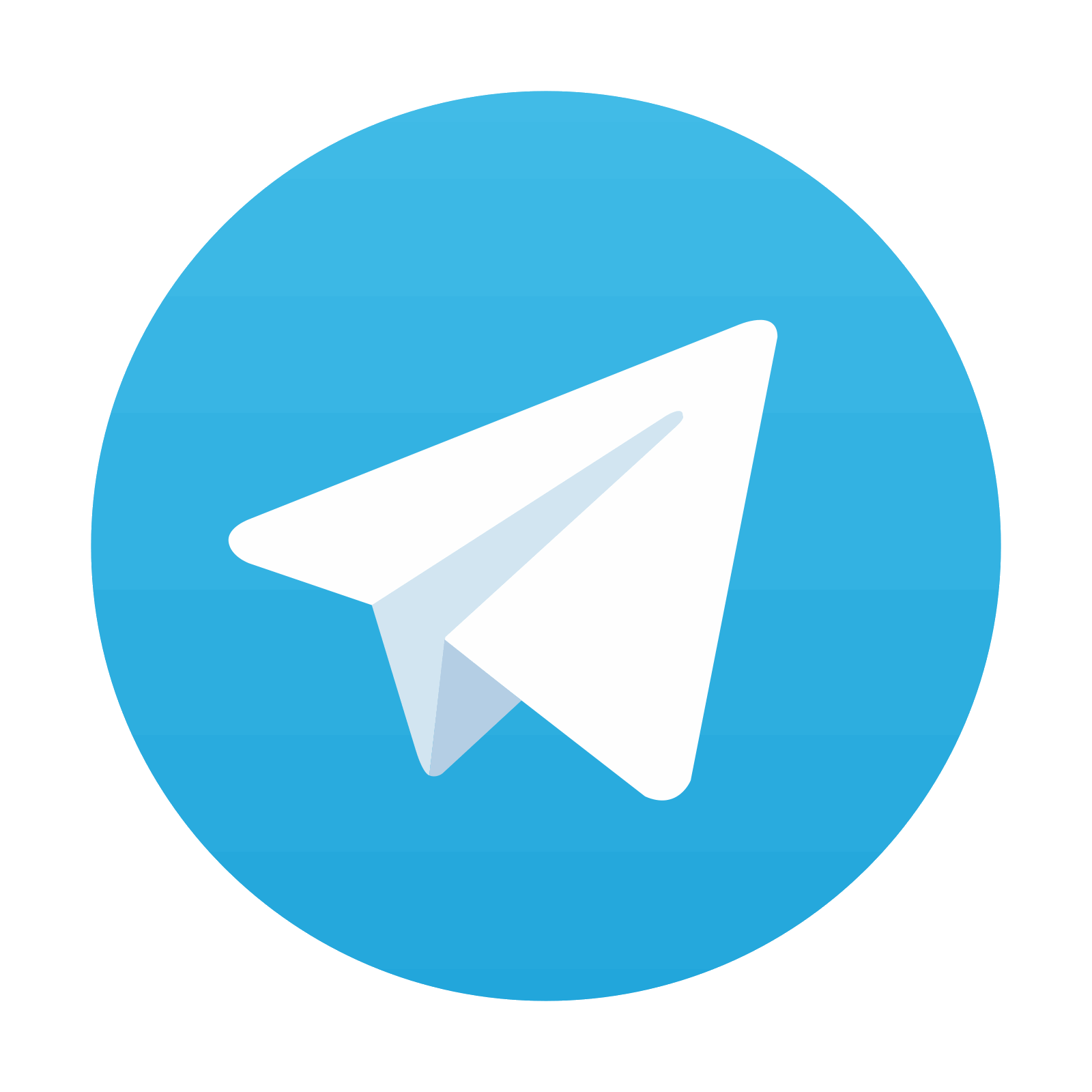
Stay updated, free articles. Join our Telegram channel
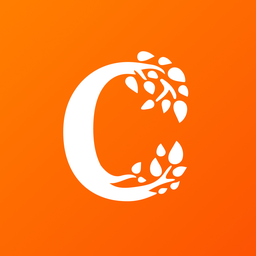
Full access? Get Clinical Tree
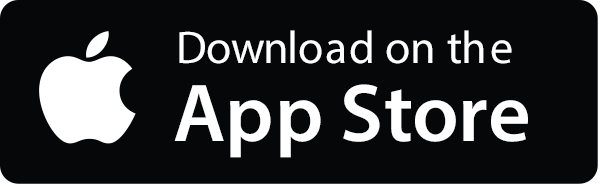
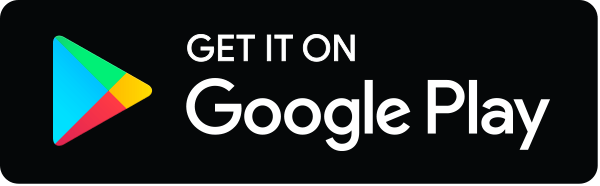
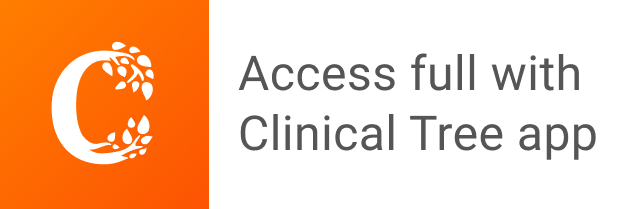